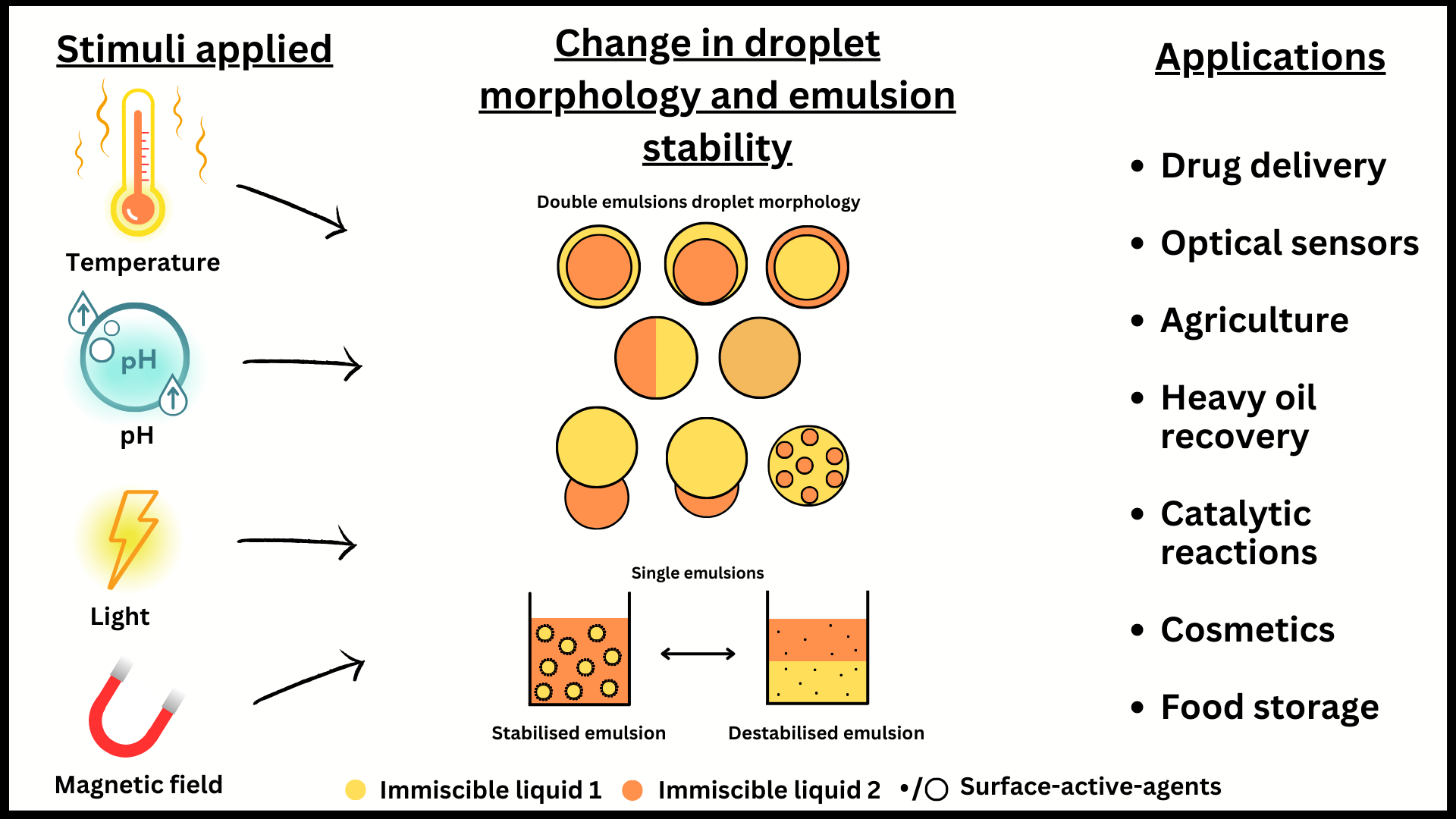
Abstract
Smart responsive emulsions, stabilised by diverse stimuli-responsive surface-active agents encompassing particles and surfactants, have gained significant attention due to their unique capability to exhibit response and recovery when triggered by external stimuli. This article explores the multifaceted potential of such emulsions, wherein changes in droplet shape, morphology and emulsion stability are induced by stimuli like pH, temperature, light, magnetic and electric fields. These smart soft materials demonstrate remarkable properties such as reversibility, low cost, facile treatment processes and easy manipulation. The ability of these emulsions to respond to environmental cues renders them invaluable in a plethora of complex applications, including drug delivery, catalytic reactions, recovery of heavy oils and their extraction, dynamic optical sensing, food storage, cosmetics and agriculture.
In this article, we discuss the principles of stimuli responsiveness in emulsions and their impact on droplet behaviour and emulsion stability when triggered by external stimuli. Developing smart and responsive soft materials can help open new horizons for innovation and integration across diverse industries, promising to revolutionise how we approach complex processes and challenges in the future.
Introduction to emulsions
Emulsions are complex thermodynamically unstable systems composed of two or more immiscible liquids with one of the phases dispersed in another phase (Fig. 1).1 The thermodynamic instability is because of high interfacial surface energy between the immiscible phases due to the small size of droplets. When interfacial tension is close to zero, emulsions become thermodynamically stable.2–4 Therefore, according to the surface-tension theory of emulsification, surface-active agents like surfactants are utilised to reduce the interfacial tension between immiscible liquids.3 In contrast, particles stabilise the emulsions by forming a protective barrier around the interface through adsorption.
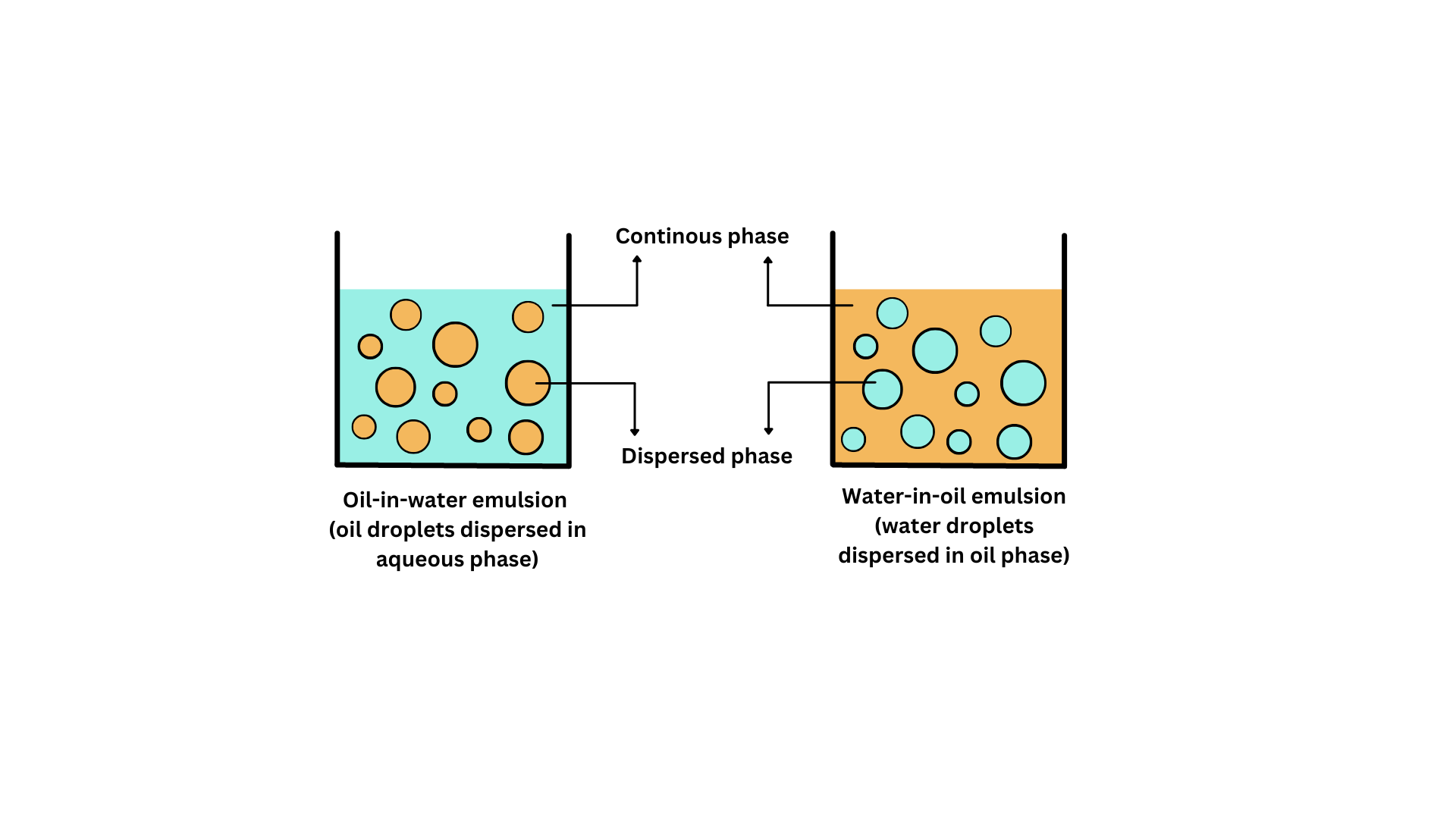
Emulsions can destabilise through multiple processes, like flocculation, creaming and sedimentation, coalescence and Oswald ripening. They minimise the total interfacial area and lower the interfacial energy via phase separation.4 Flocculation happens when small individual droplets come together and start to aggregate and form groups. Creaming and sedimentation occur due to variations in the density of liquids and separation is initiated by gravitational force. These three processes do not lead to immediate phase separation and stability can be achieved by gentle mixing to redisperse the droplets in the continuous phase. Coalescence happens when two or more droplets fuse together to form a big droplet. In this case, simple mixing does not help in regaining stability. Oswald ripening is a phenomenon where small droplets dissolve because they are more soluble than larger ones. As time goes on, the dissolved molecules condense onto the larger drops, making them even bigger. This happens because of the difference in solubility between small and large droplets, causing the small ones to gradually disappear and contribute to the growth of the larger ones. This process ultimately leads to phase separation.5
Emulsions are formed by applying high mechanical shear stress to the system by methods like sonication, high-speed homogenisation, high-pressure homogenisation or vortex mixing to break the bulk phase dispersion into tiny droplets, which are suspended in the continuous phase.6,7 Other advanced techniques, like microchannel and microfluidic emulsification, are used to prepare highly structured, monodispersed emulsions with precise control over the droplet size.6,8,9
Pickering emulsions
Pickering emulsions are a complex mixture of two or more immiscible phases stabilised by solid particles instead of surfactants. Emulsions stabilised by solid particles instead of molecular surfactants offer a host of advantages, including reduced toxicity, cost-effectiveness, long-lasting emulsion stability and the ability to recycle particles for stabilising other emulsions. This makes them increasingly favoured in diverse fields such as pharmaceutical engineering, cosmetology, agriculture, optical sensing, food storage and oil recovery.10
The solid particles in Pickering emulsions exhibit a remarkable ability to interact with multiple immiscible phases simultaneously due to their tuneable functionality. As a result, these emulsions possess several unique properties, such as:
· Irreversible adsorption: Because of the enormous desorption energy of particles at the interface, which is a thousand times greater than the system's thermal energy, particles have firm adsorption at the interfacial region, leading to enhanced stability.4,6 Through innovative composition and design, these particles can be tailored to effectively resist emulsion deformation due to their robust and strong attachment at the interface.
· Biocompatibility: Biocompatible particles for Pickering emulsions can be derived from natural resources and then functionalised and modified to ensure favourable interactions with the emulsion's interface. This adaptability allows them to be compatible with a wide range of biological systems, making them suitable for various applications in the biomedical and pharmaceutical fields.4,6
· Stimuli-responsiveness: Systems can be designed to respond to external stimuli like pH, temperature, salt concentration, magnetic or electric field, light and ionic strength. The stabilising particles can have additional properties that can be triggered or altered by specific external stimuli, leading to reversible changes in the emulsion's behaviour.4
The particles stabilising the oil-water interface must be small in size (nanometer or submicron size) to stabilise emulsion droplets with the size of a few microns or even greater up to a few millimetres.10 Particle wettability, expressed mainly by contact angle (θow), plays a significant role in stabilising the system at the oil-water interface.4,6,10 The emulsion type, oil-in-water (o/w) or water-in-oil (w/o), is decided based on the contact angle at the interface. A θow greater than 90 degrees signifies that the particle is more hydrophobic, and a θow less than 90 degrees signifies that the particle is more hydrophilic. If the particle is more hydrophobic in nature, it will tend to interact more with the oil phase, resulting in the formation of w/o type emulsion. Conversely if the particle is more hydrophilic in nature, formation of o/w type emulsion is favoured (Fig. 2).7
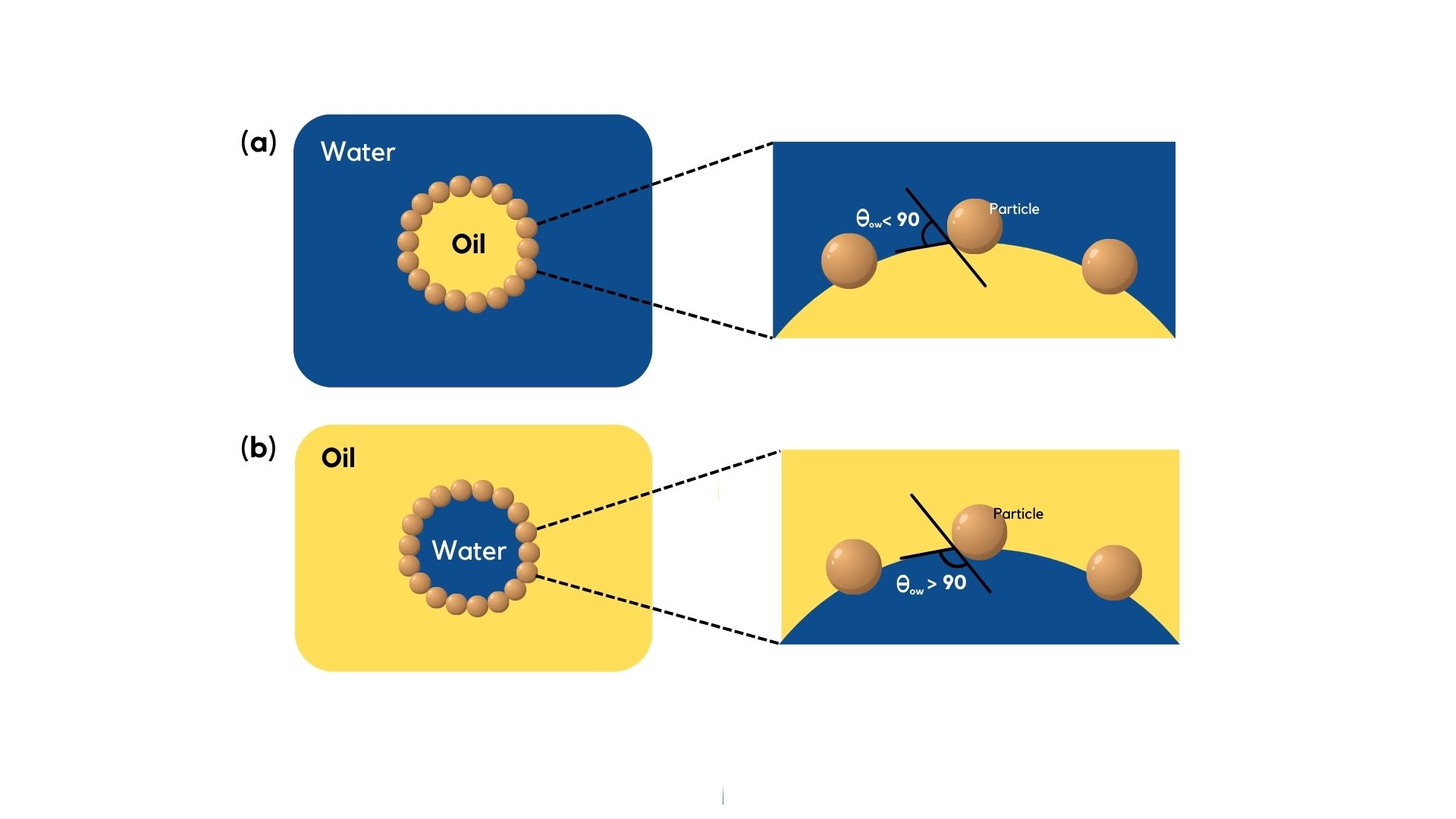
Multiple or multiphase emulsions
Multiple emulsions are complicated multiphase systems in which large droplets contain smaller drops. The complexity increases as layers of multiphase emulsion increase (i.e., more droplets within droplets). The emulsion system can have different combinations like o/w/o,11 o/o/w,12 w/o/w,13 w/o/o/w14 and o/o/o.15 This system results in multi-compartmentalization and creates microspheres with the capacity to encapsulate different chemical substances with different properties like polarity, functionality, solubility and pH. Multicompartment drops have an important role in the isolation, protection and controlled release of substances.16 Maintenance of thermodynamic stability in multiphase emulsions is quite difficult and can lead to the coalescence of inner droplets, thereby nullifying the ability to compartmentalise.17 Thus, stabilisation of multiphase emulsion with solid particles is a better option as it helps in the formation of strong and rigid films at the interface.17,18
Multiple emulsions can be prepared commonly via four ways and can require more than one emulsifier based on the number and type of interfaces present (Fig. 3).
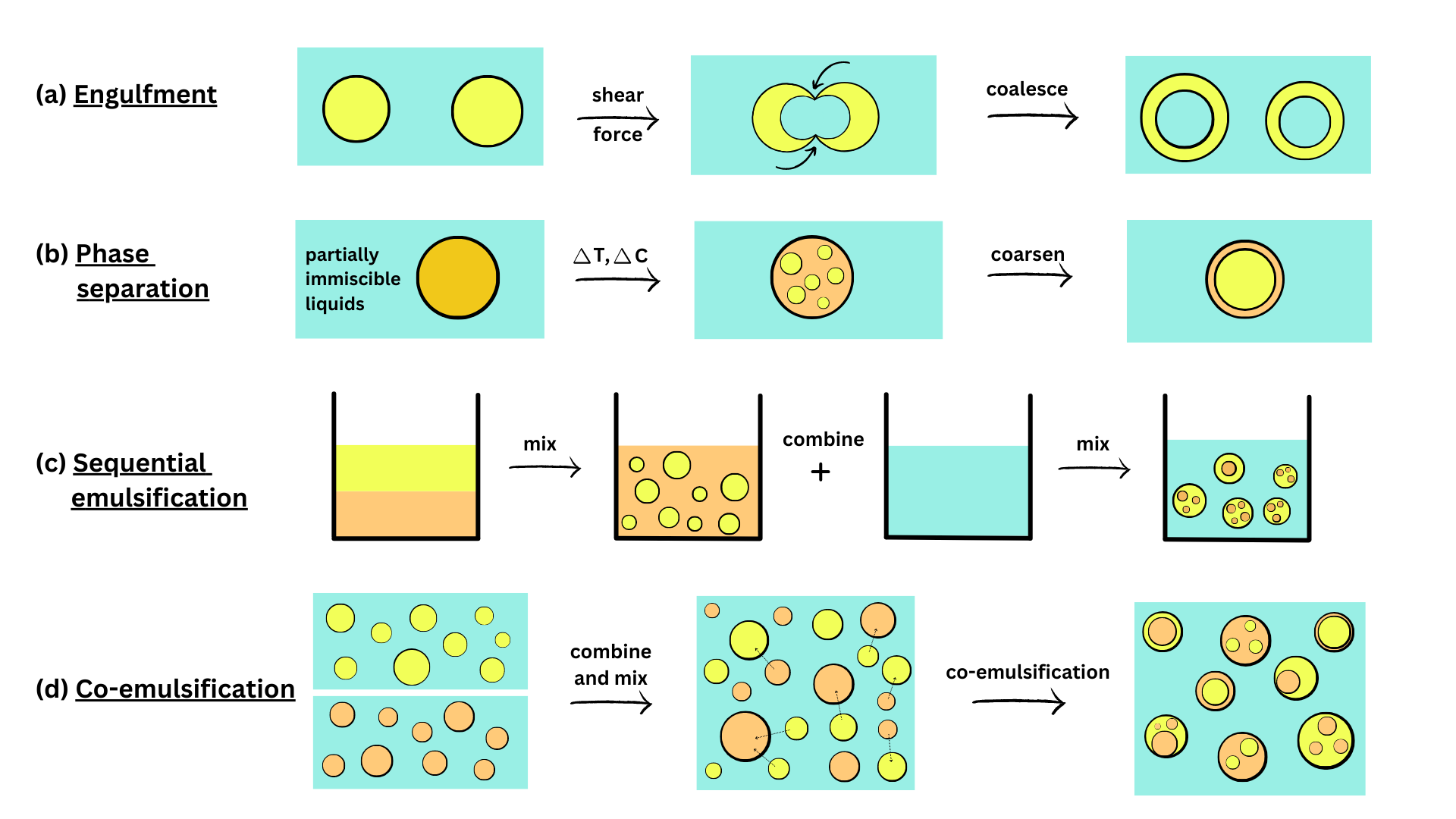
· Engulfment is the process by which continuous-phase liquid enters the dispersed phase (inside the droplet) and forms a small droplet by applying a large amount of shear force.16 This is a one-step emulsification process.
· Phase separation involves preparing a precursor emulsion with partially miscible liquids in the dispersed phase. A change in thermodynamic state (e.g., temperature, concentration) triggers phase separation, leading to the arrangement of two distinct phases within the droplets.16,17 This is also a single-step emulsification process.
· Sequential emulsification is achieved when an emulsion is mixed with an immiscible liquid so that the original emulsion forms the dispersed phase within the new continuous phase. It comes under the category of a two-step emulsification process.16,17
· Co-emulsification is a process of mixing two or more stabilised emulsions with the same continuous phase but different dispersed phases so that multiple interfaces are created.16,17 This is a two-step emulsification process.
Stimuli-responsive emulsions
Traditionally, surfactant or particle emulsifiers were chosen to maximise the long-term stability of an emulsion. The ability to manipulate the stability or structure of an emulsion is attractive, however, for some applications. These applications require, for example, controlled release of encapsulated ingredients or recovery of components from the emulsion. The development of stimuli-responsive emulsifiers has made it possible to formulate responsive emulsions. The emulsifiers may be sensitive to chemical changes, such as a change in pH or to physical stimuli such as heat, magnetic fields or light.
pH-responsive emulsions
Introducing pH-sensitive functional groups such as carboxy, amine or phenolic groups and other charged moieties onto the surface-active agents creates a new class of emulsifiers. These pH-sensitive emulsifiers exhibit dynamic behaviour in response to changes in the surrounding pH, influenced by their isoelectric point. When the environmental pH is adjusted by adding acid or base to the dispersing medium, the pH-sensitive emulsifiers undergo reversible deprotonation and protonation, effectively altering their adsorption capacity at the interface. As a consequence, the stabilisation ability of the emulsions is influenced, leading to intriguing changes in their morphology and behaviour. Moreover, pH-sensitive particles can also be engineered through different approaches. One method involves covalently attaching amphiphilic molecules to the particle surfaces, imparting pH-responsive characteristics. Alternatively, inorganic composites with pH-sensitive polymers can be directly synthesised, or hydrophilic charged particles can be utilized to achieve pH-sensitive properties.19
Binks and his group developed an eco-friendly method to temporarily stabilise emulsions using a smart surfactant synthesised from alkyl trimethylammonium bromides.20 The surfactant effectively stabilised the oil-water interface under neutral and basic pH conditions, but its stabilising ability was compromised in acidic pH environments, leading to emulsion instability. It was observed that after demulsification, the surfactants mobilised to the aqueous phase without contaminating the oil phase. The smart surfactants can be recovered again by altering pH in the aqueous phase, making the process recyclable and sustainable.20
Temperature-responsive emulsions
Temperature stimulus offers a powerful means to manipulate emulsion droplet morphology and stability without altering the chemical composition of the system. Thermal-responsive emulsifiers are engineered through the surface grafting of polymers like poly (N-isopropyl acrylamide) (PNIPAM) and poly[2-(dimethylamino)ethyl methacrylate] (PDMAEMA) to exhibit distinct temperature-sensitive behaviours for reversible emulsion stability and droplet morphology changes.21
The solubility of surfactants also plays an essential role in emulsion behaviour. Ionic surfactants for instance display increased hydrophilicity above their Kraftt point (the minimum temperature at which surfactant can form micelles) as all the surfactant molecules gather together and form micelles which are more soluble in water. In contrast, non-ionic surfactants become more hydrophobic due to the dehydration of their hydrophilic groups at higher temperatures.19 These shifts in surfactant behaviour influence the protective film surrounding the emulsion droplets. Heating weakens this protective film by reducing adsorption capacity at the interface. It also increases the likelihood of droplet collision and demulsification rises, affecting the overall stability of the emulsion.
Alternatively, emulsions not stabilised by thermo-responsive emulsifiers can also show changes in morphology or stability with changes in temperature due to their composition. For example, Zarzar et al. developed temperature-responsive oil-in-oil-in-water (o/o/w) double emulsions containing hydrocarbon and fluorocarbon oils stabilised by a non-thermo-responsive surfactant.22 It was observed that the two compartments containing hydrocarbon and fluorocarbon oil mix together and become miscible above the upper critical solution temperature (UCST) and form an oil-in-water (o/w) emulsion. However, as the temperature falls below the UCST, the double compartments re-emerge, forming an o/o/w emulsion showcasing a reversible behaviour.22
Magnetic field-responsive emulsions
Magnetic field stimulation is a non-invasive and powerful trigger that maintains the chemical composition of the emulsion system. It is widely employed for efficient demulsification due to its advantages, such as irreversible adsorption, low toxicity and cost-effectiveness.
To stabilise magnetic responsive emulsions, stabilisers are magnetic amphiphilic molecules or metal oxide nanoparticles like ferrous oxide, cobalt and nickel particles which possess high saturation ferromagnetic properties. Alternatively, self-assembled amphiphilic magnetic polymer micelles can stabilise the biphasic interface. Through the application of an external magnetic field, the emulsion droplets can be easily manipulated and reoriented, resulting in changes in their structure and overall stability. When subjected to an external magnetic field, rapid demulsification occurs, and the nanoparticles can be efficiently recycled for use in subsequent emulsions.19
Jinben Wang et al. achieved a significant breakthrough by developing magnetically switchable emulsions using 3-aminopropyltriethoxysilane-coated Fe3O4-SiO2-NH2 nanoparticles as stabilisers.23 This formulation displayed the ability to completely demulsify emulsions when subjected to an external magnetic field. Impressively, the components of the emulsion were easily re-dispersed and mixed to form stable emulsions once more, only to be successfully demulsified again.23 This demonstrated the remarkable reversibility and versatility of the system.
Photo-responsive emulsions
Light stimulus holds tremendous potential for controlling the morphology and stability of photo-responsive emulsions. These emulsions are stabilised using photoactive compounds, such as photochromic molecules (e.g. azobenzene, fluorenyl, stilbene, spiropyran)24 or photosensitive surfactants and light-responsive polymers. The magic lies in their ability to undergo reversible reactions or conformational changes when illuminated with a particular wavelength of light. Upon light exposure, these photoactive components trigger a series of transformations within the emulsion. As a result, the droplet size and morphology undergo changes and alterations in interfacial properties may lead to phase inversions, where the arrangement of phases within the emulsion shifts. A key advantage of light-responsive emulsions is the ability to generate specific responses based on the characteristics of the light used - wavelength, intensity and duration of irradiation can be precisely controlled to achieve desired effects. This level of control offers exciting possibilities for tailoring emulsion behaviour and properties for specific applications.19
Bing Liao et al. achieved a remarkable feat by creating a three-phase emulsion system comprising two different oils (hexane and perfluoro octane) and water.25 The emulsion was stabilised using a combination of fluoro surfactant and a light-responsive surfactant called 2-(4-(4-butylphenyl) diazenylphenoxy) ethyl trimethylammonium bromide (C4AZOC2TAB). Through the application of UV blue light irradiation, the researchers successfully induced a phase inversion of their double emulsion, transitioning from an F/H/W (fluoro/hexane/water) configuration to a Janus (bilayer) structure and finally to a H/F/W (hexane/fluoro/water) configuration. The key to this achievement is in the light-responsive surfactant C4AZOC2TAB, which displayed an intriguing increase in surface tension at the hexane-water interface when irradiated with UV light. This phenomenon resulted in a photo-induced morphological change in the emulsion, enabling the precise control of its structure and behaviour.25
Potential applications
The stimuli-responsive emulsions find various applications in a wide range of fields. Some areas where stimuli-responsive emulsion systems would be of particular value include drug delivery,26 catalytic reactions,27 the food industry,28 oil recovery,29 dynamic optical sensing30 and many more. Stimuli-responsive emulsions can offer controlled drug release under specific conditions, such as changes in pH, body temperature or enzymatic activity. This targeted behaviour enhances drug effectiveness while minimising side effects. This enables continuous and tailored medication administration, reducing adverse effects and increasing treatment effectiveness.31 Cosmetics and personal care items can employ emulsions that react to changes in temperature, pH or light.32
In the food industry, emulsions that respond to stimuli can improve the stability and sensory qualities of food items. For instance, emulsions that react to temperature changes during cooking or processing might enhance the end product mouthfeel and texture. Emulsions responsive to external stimuli like light or magnetic fields can be used in biomedical imaging techniques for contrast enhancement or targeted imaging of specific areas.33 By aiding the flow of oil, emulsions that alter their rheological characteristics in response to certain circumstances in oil reservoirs can be utilised to enhance oil recovery procedures.21 Emulsions that react to environmental cues can help in the removal of contaminants or pollutants, enabling focused and effective remediation procedures. These are only a few instances of how stimuli-responsive emulsion systems could be used. The adaptability of these emulsions offers numerous opportunities for innovation and impactful usage in a variety of sectors.
Conclusions
Smart and responsive emulsions, stabilised by diverse stimuli-responsive surface-active agents, hold immense promise across various industries. Their ability to exhibit response and recovery when triggered by external stimuli such as pH, temperature, light and magnetic or electric fields opens up new horizons for innovation and integration. These emulsions demonstrate remarkable properties, including reversibility, low cost, facile treatment processes, and easy manipulation. Their applications span drug delivery, catalytic reactions, oil recovery, food storage, cosmetics and agriculture. With the potential to revolutionise complex processes and challenges in the future, stimuli-responsive emulsions offer versatile solutions for diverse fields, paving the way for exciting advancements and improved functionalities in various products and processes.
References
1. Jiao, J.; Rhodes, D. G.; Burgess, D. J. J. Colloid Interface Sci. 2002, 250 (2), 444–450. https://doi.org/10.1006/jcis.2002.8365.
2. Kabalnov, A. Curr. Opin. Colloid Interface Sci. 1998, 3 (3), 270–275. https://doi.org/10.1016/S1359-0294(98)80071-X.
3. Kale, S. N.; Deore, S. L. Systematic Rev. Pharmacy 2016, 8 (1), 39–47. https://doi.org/10.5530/srp.2017.1.8.
4. Sun, Z.; Yan, X.; Xiao, Y.; Hu, L.; Eggersdorfer, M.; Chen, D.; Yang, Z.; Weitz, D. A. Particuology 2022, 64, 153–163. https://doi.org/10.1016/j.partic.2021.06.004.
5. Hu, Y. T.; Ting, Y.; Hu, J. Y.; Hsieh, S. C. J. Food Drug Anal. 2017, 25 (1), 16–26. https://doi.org/10.1016/j.jfda.2016.10.021.
6. Zhao, H.; Yang, Y.; Chen, Y.; Li, J.; Wang, L.; Li, C. Chem. Eng. Sci. 2022, 248, 117085. https://doi.org/10.1016/j.ces.2021.117085.
7. Brito, F.; Carvalho-guimar, D.; Correa, K. L.; Souza, T. P. De. Pharmaceuticals 2022, 15, 1413–1434. https://doi.org/https://doi.org/10.3390/ph15111413.
8. Sugiura, S.; Nakajima, M.; Seki, M. J. Am. Oil Chem. Soc. 2002, 79 (5), 515–519. https://doi.org/10.1007/s11746-002-0517-6.
9. Maan, A. A.; Nazir, A.; Khan, M. K. I.; Boom, R.; Schroën, K. J. Food Eng. 2015, 147, 1–7. https://doi.org/10.1016/j.jfoodeng.2014.09.021.
10. Chevalier, Y.; Bolzinger, M. A. Colloids Surf. A Physicochem. Eng. Asp. 2013, 439, 23–34. https://doi.org/10.1016/j.colsurfa.2013.02.054.
11. Bernewitz, R.; Schmidt, U. S.; Schuchmann, H. P.; Guthausen, G. Colloids Surf. A Physicochem. Eng. Asp. 2014, 458 (1), 10–18. https://doi.org/10.1016/j.colsurfa.2014.01.002.
12. Seo, M.; Paquet, C.; Nie, Z.; Xu, S.; Kumacheva, E. Soft Matter 2007, 3 (8), 986–992. https://doi.org/10.1039/b700687j.
13. Okochi, H.; Nakano, M. Adv. Drug Deliv. Rev. 2000, 45 (1), 5-26. https://doi.org/10.1016/S0169-409X(00)00097-1
14. Zheng, W. Int. J. Pharm. 2009, 374 (1–2), 90–95. https://doi.org/10.1016/j.ijpharm.2009.03.015.
15. Elkharraz, K.; Ahmed, A. R.; Dashevsky, A.; Bodmeier, R. Int. J. Pharm. 2011, 409 (1–2), 89–95. https://doi.org/10.1016/j.ijpharm.2011.02.029.
16. Sheth, T.; Seshadri, S.; Prileszky, T.; Helgeson, M. E. Nature Res. 2020, 5, 214–228. https://doi.org/10.1038/s41578-019-0161-9.
17. Zhao, H.; Yang, Y.; Chen, Y.; Li, J.; Wang, L.; Li, C. Chem. Eng. Sci. 2022, 248, 117085-117099. https://doi.org/10.1016/j.ces.2021.117085.
18. Chevalier, Y.; Bolzinger, M. A. Colloids Surf. A Physicochem. Eng. Asp. 2013, 439, 23–34. https://doi.org/10.1016/j.colsurfa.2013.02.054.
19. Ge, X. hui; Mo, L.; Yu, A.; Tian, C.; Wang, X.; Yang, C.; Qiu, T. Chin. J. Chem. Eng. 2022, 41, 193–209. https://doi.org/10.1016/J.CJCHE.2021.11.002.
20. Pei, X.; Zhang, S.; Zhang, W.; Liu, P.; Song, B.; Jiang, J.; Cui, Z.; Binks, B. P. Angew. Chem. Int. Ed. 2021, 60 (10), 5235–5239. https://doi.org/10.1002/anie.202013443.
21. Tang, J.; Quinlan, P. J.; Tam, K. C. Soft Matter 2015, 11 (18), 3512–3529. https://doi.org/10.1039/c5sm00247h.
22. Zarzar, L. D.; Sresht, V.; Sletten, E. M.; Kalow, J. A.; Blankschtein, D.; Swager, T. M. Nature 2015, 518 (7540), 520–524. https://doi.org/10.1038/nature14168.
23. Yang, H.; Hou, Q.; Wang, S.; Guo, D.; Hu, G.; Xu, Y.; Tai, J.; Wu, X.; Yu, D.; Wang, J. Chem. Comm. 2018, 54 (76), 10679–10682. https://doi.org/10.1039/c8cc04811h.
24. Eastoe, J.; Vesperinas, A. Soft Matter 2005, 1 (5), 338–347. https://doi.org/10.1039/b510877m.
25. Jia, K.; Zhang, X.; Zhang, L.; Yu, L.; Wu, Y.; Li, L.; Mai, Y.; Liao, B. Langmuir 2018, 34 (38), 11544–11552. https://doi.org/10.1021/acs.langmuir.8b02456.
26. Zhang, Q.; Zhao, Q.; Zhu, B.; Chen, R.; Zhou, Y.; Pei, X.; Zhou, H.; An, H.; Tan, Y.; Chen, C. Int. J. Biol. Macromol. 2023, 244, 125393-125402. https://doi.org/10.1016/J.IJBIOMAC.2023.125393.
27. Chen, Z.; Zhao, C.; Ju, E.; Ji, H.; Ren, J.; Binks, B. P.; Qu, X. Adv. Mater. 2016, 28 (8), 1682–1688. https://doi.org/10.1002/adma.201504557.
28. Dickinson, E. Curr. Opin. Colloid Interface Sci. 2010, 15 (1–2), 40–49. https://doi.org/10.1016/J.COCIS.2009.11.001.
29. Wang, X.; Shi, Y.; Graff, R. W.; Lee, D.; Gao, H. Polymer 2015, 72, 361–367. https://doi.org/10.1016/J.POLYMER.2014.12.056.
30. Chen, L.; Li, Y.; Fan, J.; Bisoyi, H. K.; Weitz, D. A.; Li, Q. Adv. Opt. Mater. 2014, 2 (9), 845–848. https://doi.org/10.1002/adom.201400166.
31. Harman, C. L. G.; Patel, M. A.; Guldin, S.; Davies, G. L. Curr. Opin. Colloid Interface Sci. 2019, 39, 173–189. https://doi.org/10.1016/J.COCIS.2019.01.017.
32. Patravale, V. B.; Mandawgade, S. D. Int. J. Cosmet. Sci. 2008, 30 (1), 19–33. https://doi.org/10.1111/j.1468-2494.2008.00416.x.
33. Medeiros, S. F.; Santos, A. M.; Fessi, H.; Elaissari, A. Int. J. Pharm. 2011, 403 (1–2), 139–161. https://doi.org/10.1016/j.ijpharm.2010.10.011.