Abbreviations
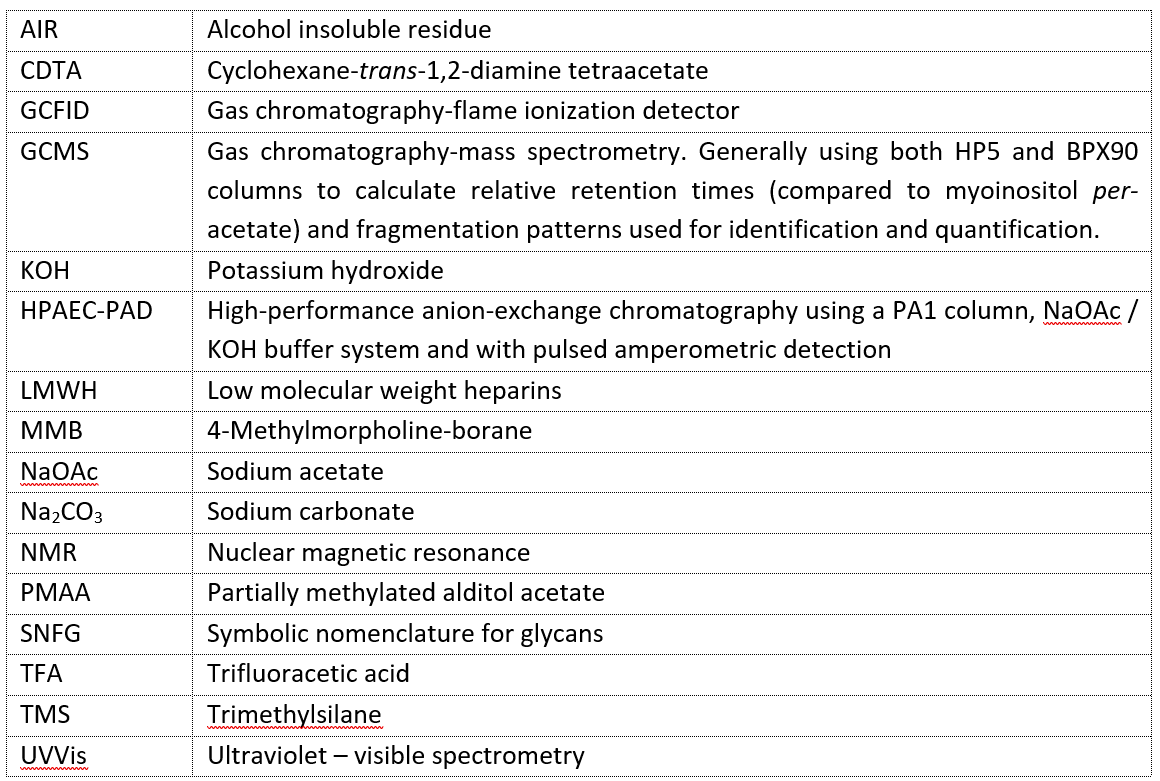
Introduction
Polysaccharides, complex high molecular weight carbohydrates, are arguably the most important class of biopolymers; but then we would say that. The evolution and subsequent dominance of humans has been possible only through their ability to utilise polysaccharides. A bold claim, but starch (a polymer of α-linked D-Glc) is the main source of digestible energy in humans, and is a major component of the diet, particularly among agricultural societies that developed from hunter-gatherers. The domestication and breeding of starch-rich crops such as maize, rice and wheat for human consumption, and rapidly growing animal feedstocks enriched in carbohydrates, has played a major role in the differentiation of humans from all other animals.
Consider next cellulose, a β-D-Glc-(1→4) linear polymer (Fig. 1) that is (mostly) unbranched (more on that later, see Fig. 2). Let us remind ourselves that while we can’t digest cellulose as we lack the enzymatic machinery (that is the domain of fungi and termites), it differs in structure from starch by only the orientation of the glycosidic linkage. One linear glucose-repeat polymer is a generally amorphous mostly water-soluble food, the other displays remarkable solubility resistance and significant crystalline character providing other key human survival components in the form of building materials as well as a renewable and carbon-neutral energy source. Forget your soulless battery-powered car; if you want a truly long-term sustainable carbon-neutral option go tree fired steam.
Another water insoluble polysaccharide is the cellulose of the sea, chitin; also present in fungi and insects. Found in nature as a linear repeating β-D-GlcNAc-(1→4) polymer, generally 80% N-acetylated, this amide linkage may be (mostly) hydrolysed to chitosan producing a polysaccharide that has much greater solubility and therefore utility in subsequent chemical modifications. While the literature abounds with the chemistry of chitin and derivatives,1 commercial utility is limited to filter aids and relatively low-value food additives: to our knowledge a high value application remains elusive.

And finally, one must not forget that medicinally ubiquitous polysaccharide that is spray coated onto the inside of every blood collection syringe, is administered wholesale in surgeries and is a therapeutic in its own right: heparin. Where would we be without this life saving anticoagulant3 squeezed from the guts of pigs4 soon to celebrate its 90th year as a therapeutic. A remarkable life-saving drug with a myriad of medical applications that must pose quite a dilemma for the average card-carrying vegan blood donor.
A theme that may have become apparent in reading this introduction, is while optimised configurations and constituents in a polysaccharide are proposed (and oft assumed), these describe only most of the diversity found in nature. Reconsider heparin, an (always!) linear polysaccharide comprised of a series of repeating disaccharide motifs that are variously sulfated and of a range of chain lengths. The eight major disaccharide units found, synthesised in a non-template driven manner leads to the analytically terrifying concept that potentially every molecule in a naturally occurring population may be different. Even in a chain of eight sugars (four disaccharide building blocks) there are > 4000 possible arrangements (minor (but extremely important biologically) 3-O-sulfated moieties excused). Not trivial to deconvolute for even the most adept analytical chemist.5
In this summary we unapologetically introduce only the techniques applied in our laboratory for the identification and quantification6 of sugar moieties in complex samples. The level of technical detail provided is sufficient to indicate our choice of optimum technique, but does assume significant familiarity with the field and assumes the reader will pursue the literature to garner the specific methodology required to successfully effect the transformations described. A myriad of other approaches, variables and considerations, particularly for specialist carbohydrate moieties or the application of a peculiar technique,7, 8 can be found in the literature.9
While huge advances have been made in the application of solution NMR for the characterisation of complex carbohydrates,10 this requires the species to be soluble, may require higher temperature to ensure no gel character, and if multiple combinations of different classes of polysaccharide are present (that is oft the case) is extremely time consuming to deconvolute. The complex 1H NMR spectra recorded due to highly overlapped “sugar regions”, where often only the anomeric signal is immediately diagnostic, has made the advances in sensitivity and resolution of 2D NMR particularly useful. However, the vagaries of 2D peak intensities to quantify sugar contributions11 is limited in determining how much of each sugar residue is present,12 even if the exact nature of it can be ascertained.
Therefore, the chemical approaches described herein represent a combination of the state of the art, and those techniques that are tried and true based on hard won experience.
Constituent sugar analysis
Constituent sugar analysis can be as simple as how much total carbohydrate is present, through to the individual sugar arrangement, branchpoint, anomeric orientation and chirality in a polysaccharide. There are a range of colorimetric assays to determine total carbohydrate, uronic acids, hexosamines and other specific types of sugar.13
Identifying and quantifying the sugars that a polysaccharide is composed of requires hydrolysis, usually using acid, to cleave the polysaccharide to its component monosaccharides. While this sounds trivial there are a multiplicity of factors that need to be taken into account, including the relative susceptibility of different glycosyl linkages to cleavage and the stability of the sugars released, as well as sample type (soluble versus insoluble) and matrix effects (e.g. what other non-sugar components are present). Even apparently minor differences in methodology can affect the results obtained; acid hydrolysis can be achieved using a heating block at 121°C for 1 h, but the data is more consistent when using autoclaving to accomplish hydrolysis.14
There are a wide range of different methods available to determine the individual sugars that comprise any given polysaccharide. There are three methods we use in our laboratory. GC-MS analysis following hydrolysis with TFA and derivatisation to alditol acetates is used to determine neutral sugars, while GC-MS of TMS derivatives following methanolysis enables detection of both neutral and acidic sugars.15 For routine constituent sugar analyses we now use HPAEC after hydrolysis of the polysaccharides to their component monosaccharides.16 This method has the advantage of measuring neutral and acidic sugars in a single chromatographic run without requiring time-consuming derivatisations.
These methods do not, however, hydrolyse cellulose which requires sulfuric acid to depolymerise to monosaccharides.17 This method can be used in combination with TFA hydrolysis as the first step, to hydrolyse non-cellulosic polysaccharides and sulfuric acid in the second step to hydrolyse insoluble polysaccharides, mostly comprised of cellulose.18
All of these methods are a balancing act between achieving maximum hydrolysis while minimizing destruction of the released monosaccharides. Complete recovery is almost impossible (see later note on quantitation), unless very pure polysaccharides with simple linkage compositions are being analysed.
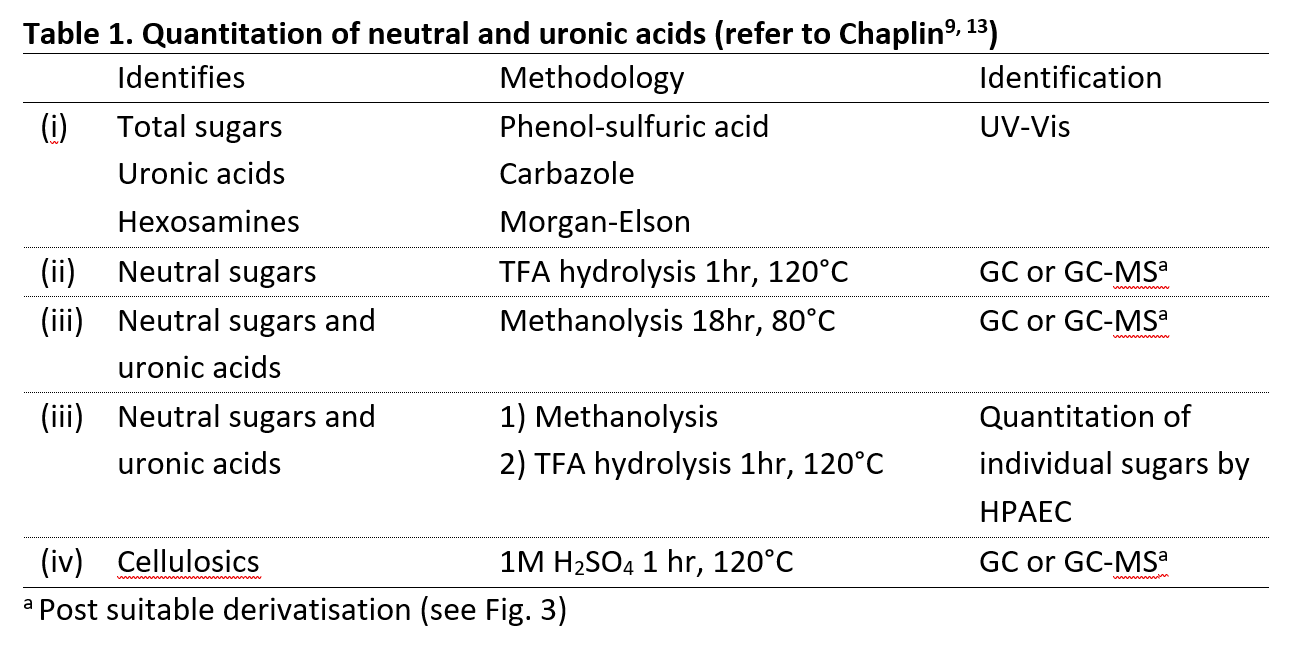
Plant cell wall analysis
While constituent sugar analysis provides an overall composition, determining how those sugars are linked in a polysaccharide to give its chemical structure introduces a whole new level of analytical complexity.
Plant cell walls are highly elaborate extracellular structures that surround each plant cell. Primary plant cell walls consist of a framework of cellulose surrounded by a matrix of pectic polysaccharides (polymers with backbones of uronic acid residues with neutral side-chains and often great structural diversity), and hemicelluloses (mostly neutral polymers with backbones of β-D-linked sugar residues, with side-chains of varying complexity). Once fully grown, the secondary cell wall provides rigidity through thickening and lignification. Understanding the complex structure of the polysaccharides in plant cell walls allows us to interpret their chemical and physical attributes and to ultimately manipulate their structures for our benefit. Thus, knowledge of cell wall polysaccharide structure can help us assess wood strength and flexibility, understand and alter fruit ripening and storage, determine how cooking changes vegetable texture and manipulate dietary polysaccharides to feed our resident microbiota for optimal health.
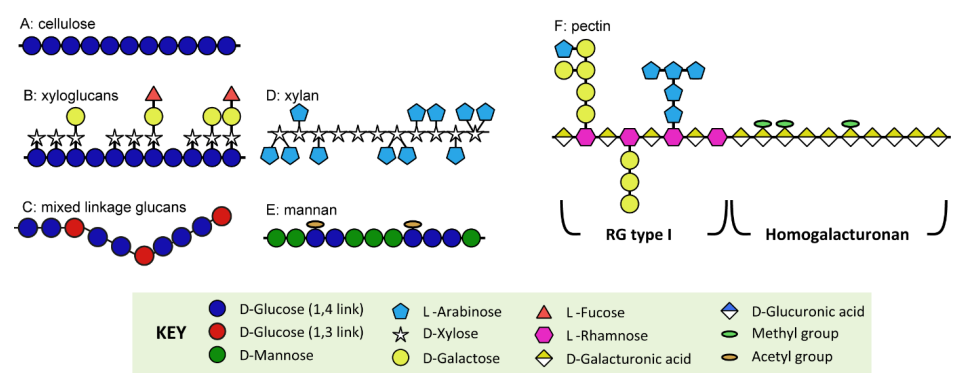
Solid state NMR can be used to study the gross molecular organisation of plant cell walls and can distinguish cell-wall polymers that have different mobilities because of their locations and interactions with other molecules.19 However, to determine the fine chemical structure of the polysaccharides that make up this complex matrix requires sequential extraction of different polysaccharide classes to solubilise them.
Sample preparation
A recognised first-step in characterising the cell-wall polysaccharides is to prepare an alcohol insoluble residue (AIR). This, generally, entails thorough de-starching of an aqueous extract of a dehydrated or frozen ground plant material,10,20 resuspension (note; not dissolution) in water and the addition of two volumes of ethanol to keep only small carbohydrates in solution. Often protease or lipid extraction treatments are applied to further maximise the carbohydrate content to be characterised. This complex mixture of carbohydrates then undergoes sequential extraction into increasingly basic solutions (Table 2) with dialysis and lyophilisation of the extracted constituents providing “pure” carbohydrate fractions for analyses.
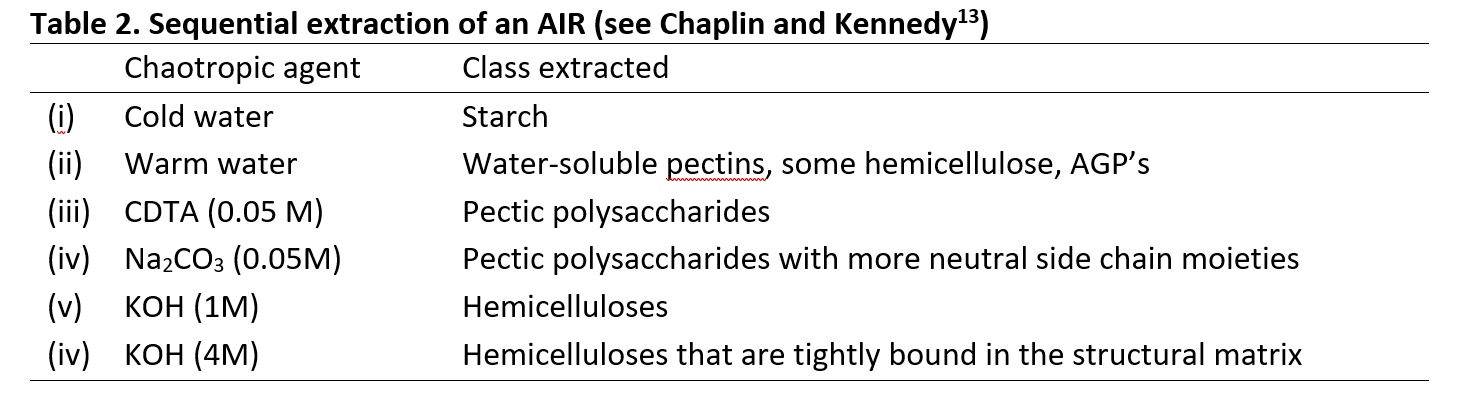
Glycosyl linkage analysis
To determine the position of the glycosidic linkages between sugars in a polysaccharide, sugars are commonly derivatised to partially methylated alditol acetates (PMAAs) to be analysed by GC-MS. These synthetic modifications (entry (i), Table 3) are shown in Fig. 3, with the example showing how 1,3-linked glucose and a 1,4-linked glucose can be distinguished.
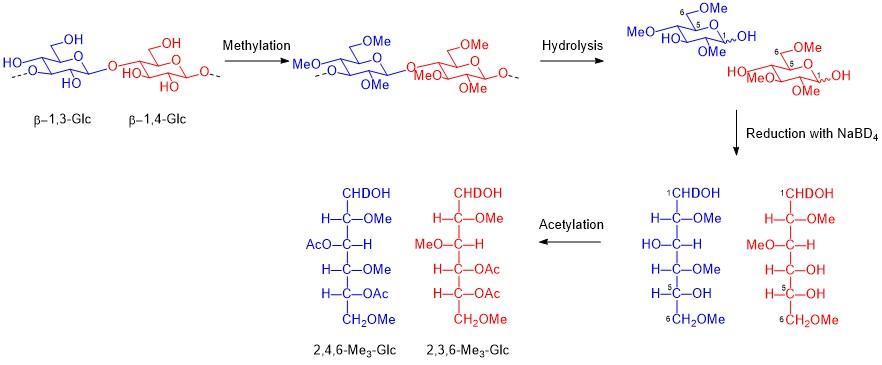
The uronic acids of acidic polysaccharides (e.g. as in pectic polysaccharides) cannot be derivatised to PMAAs directly as the carboxylic acid moiety (potentially even present as an acyl derivative) will remain, and is not amenable to GC analysis. Therefore, the carboxylic acid groups are reduced (carboxyl reduction) prior to PMAA derivatisation (entry (ii), Table 3). This is performed with a deuterated reducing agent to allow differentiation of a reduced uronic acid from the equivalent neutral sugar by MS.
When a polysaccharide contains sugars with endogenous O-methylation, CD3I is used as a methylating agent in place of CH3I to allow for MS differentiation of endogenous and synthetic methylation (entry (iii), Table 3).
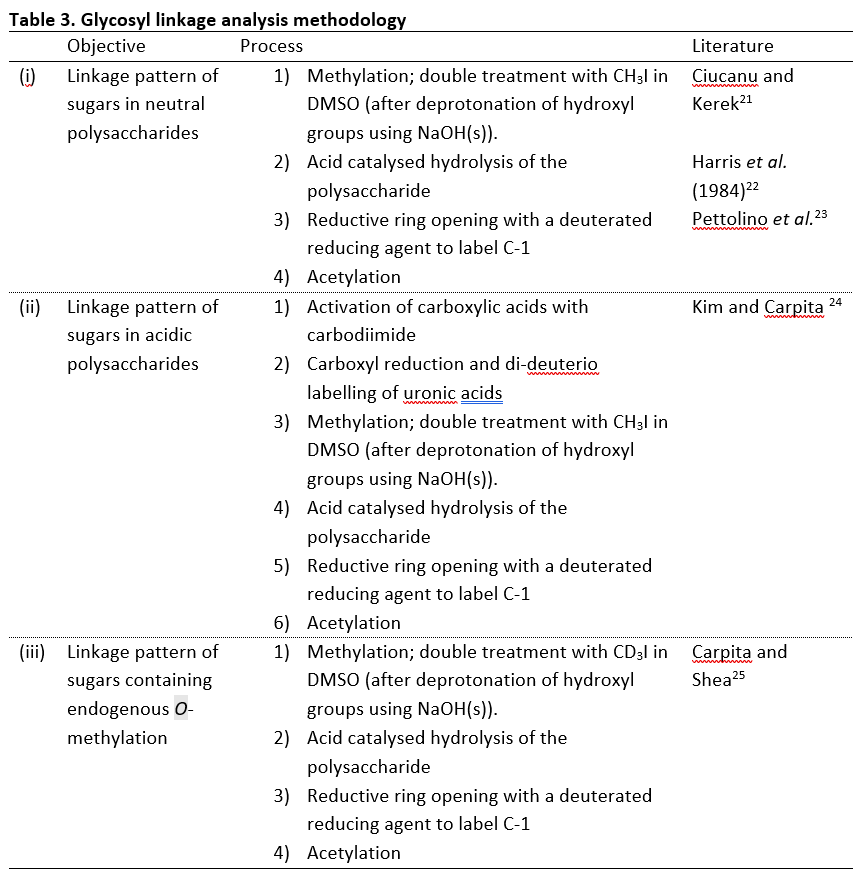
Specialist sugar analysis
An interesting complication in tackling some naturally occurring polysaccharides is heteroatom inclusion, in particular sulfation. Algae produce a diverse range of complex sulfated carbohydrates and this poses challenges in terms of solubility, reactivity to acid mediated anomeric centre hydrolysis, and in determining the position of sulfation. A series of methodologies have been developed to inform our analysis of these complex yet abundant polysaccharides (Table 4).
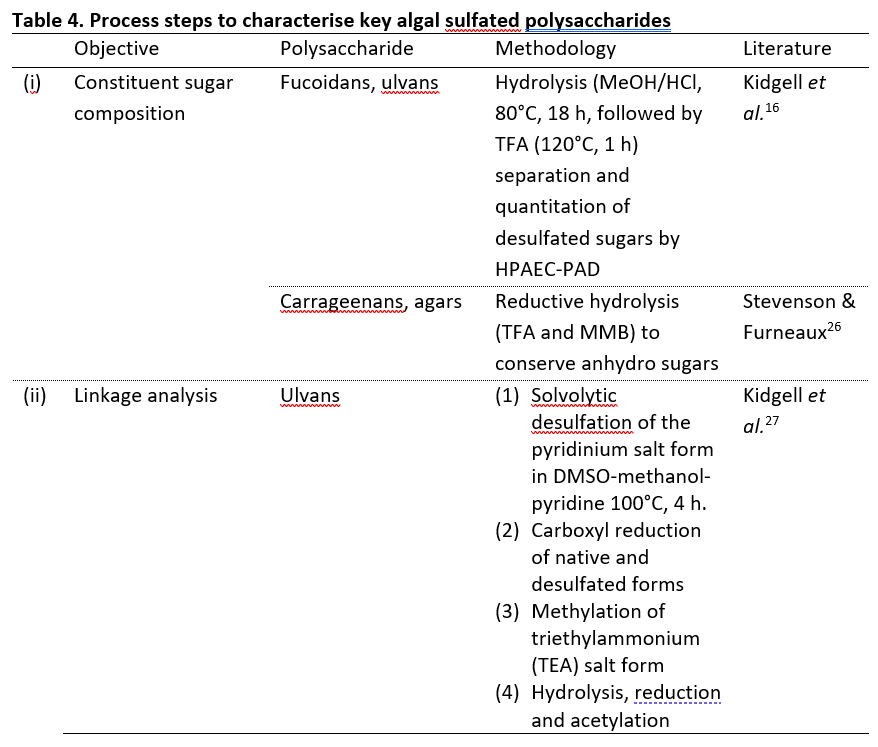
The linkage analysis described in entry (ii) Table 4 at step (2) implies undertaking methylation analysis on both the sulfated and solvolysis-mediated desulfated polysaccharide in order to provide the total functionalisation of the sugar moieties and just the sugar-linkages, respectively.
Heparin/Heparan sulfate
One could be excused for not wishing to undertake the analysis of heparin based on the observations described previously since not only does heparin contain GlcN (acetylated, sulfated or as the amine), and uronic acids, but is also variably O-sulfated – to an extent that it is the most negatively charged natural biopolymer. While heparin has been developed28 into one of the most widely prescribed pharmaceuticals, in our bodies we produce very little; but every cell does produce the very closely related heparan sulfate. Heparan sulfate has a myriad of biological functions – effectively engaged in some manner with every protein signalling activity,29 and while it is yet to be developed into a human therapeutic there is significant research ongoing in this area.30,31
This combination of functionality is particularly recalcitrant to “normal” chemical hydrolysis.
There are two main strategies for characterising heparin and heparan sulfate: top-down analysis, which examines intact chains, and bottom-up analysis, involving controlled depolymerisation of polysaccharide chains to obtain disaccharides or oligosaccharides.
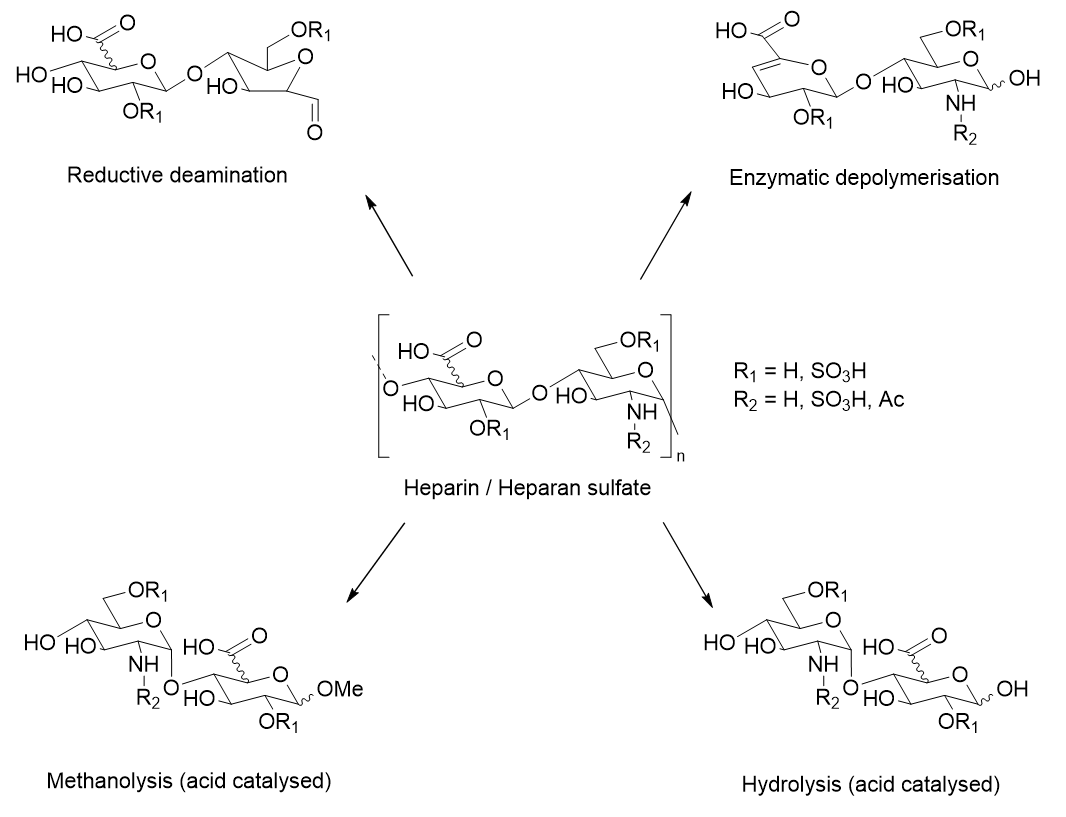
Top-Down: Analysis utilises techniques like LC-MS, MS/MS, and NMR. They permit assessment of the position of sulfate groups, uronic acid and glucosamine residues in oligosaccharides and LMWHs but face challenges with large molecules like unfractionated heparin (UFH).32
Bottom-Up: Controlled depolymerisation through enzymatic or chemical methods (see Table 5). The fragments obtained are separated and identified, either through comparison with reference standards or via MS-based identification. Each depolymerisation method has its advantages and disadvantages. For example, nitrous acid can preserve uronic acid C5 stereochemistry identifying iduronic c.f. glucuronic acid, but the disaccharide so produced requires labelling for detection. In comparison, enzymatic degradation forms a Δ4,5 double bond on the uronic acid, resulting in the loss of the C5-epimer information, but the fragments provide a chromophore for detection at 232nm.33 It is worth noting that while the Δ4,5 double bond is preserved in all the enzymatically generated disaccharides, the extinction coefficient is highly variable requiring careful quantitation to ensure accurate compositional analysis.
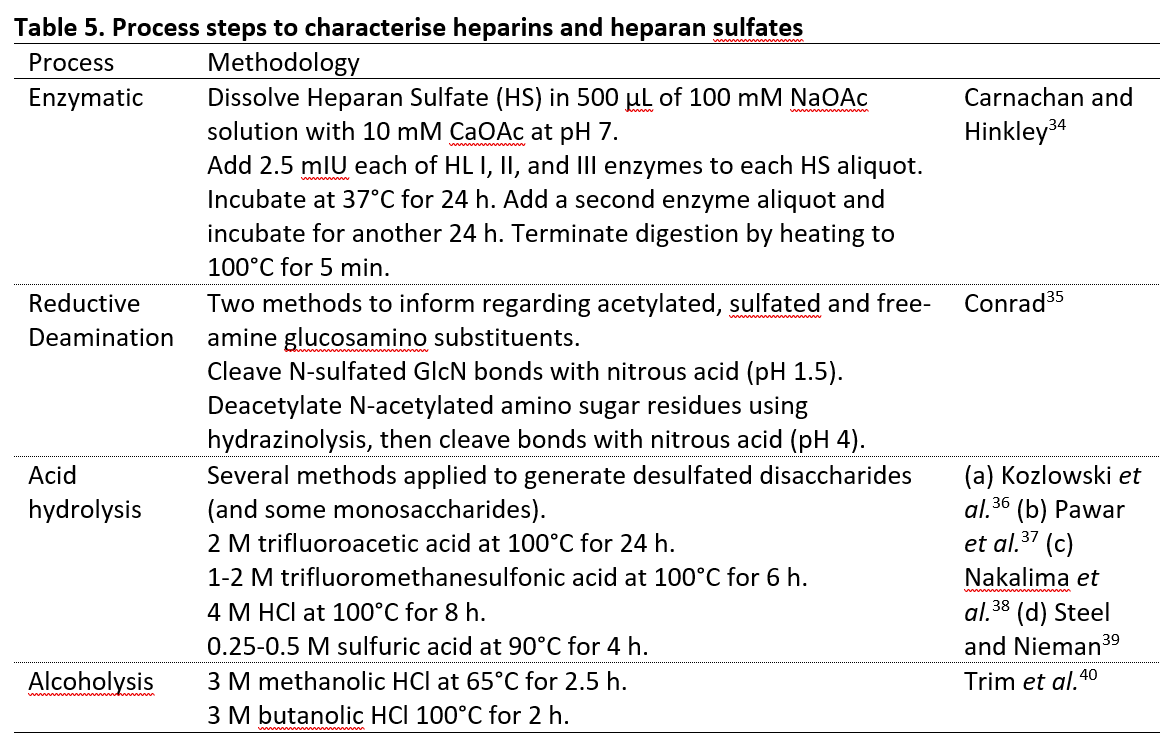
A note regarding quantitation
The analysis of carbohydrates, particularly those that report individual sugar moieties and even the permutation of linkages, relies on many individual chemical reactions going to very high yield. The initial steps of the successful separation of polysaccharide classes in a quantitative fashion based on solubility provides a degree of variability. In turn, the depolymerisation of the many different linkages (chemical or enzymatic), potentially including the reduction of uronic acids, coupled with per-methylation, anomeric centre reduction, acetylation and finally the subunit detection all require very high conversion. In addition, the detection methodologies applied must accommodate response factors for individual sugars (as well as the method utilised e.g. GCMS c.f. GCFID).
An acceptable level of precision is to record an 85% correlation between expected and observed. The analyses described here are routinely undertaken in duplicate with a < 5% variation seen between biological replicates. By way of example, a mass balance of extracting an AIR that recovered > 85% of the material after sequential extraction and dialysis steps is technically excellent. Similarly, if acid hydrolysis recovered 70% w/w of the material as sugars (excluding cellulosics) and a second Saemen hydrolysis (see acid hydrolysis entries, Table 5) indicated a further contribution of 15% w/w glucose, then the total of 85% w/w is an acceptable quantification of the raw material. Likewise, if one completes analysis of neutral sugars by HPAEC and then compares this to the “equivalent” methodology of generating PMAAs, a correlation that differs by < 15% in relative amounts and total sugar recorded is an acceptable level of precision.
Conclusions
The characterisation of carbohydrates poses a number of challenges. Assessment of complex mixtures of polysaccharides using MS or NMR provide a general tone of the overall composition. Purification of complex mixtures into more readily identified classes of polysaccharides is a complex and nontrivial step, complicated by solubility and matrix components. The polymer heterogeneity and a large range of reactive differences between sugar types, as well as the stability of depolymerised forms, requires the careful selection of suitable reagents to generate quantifiable fragments in the form of mono- or di-saccharides. This may entail several steps to distinguish relatively rare or unexpected sugar functionality – e.g. natural methylation or acetylation in the polysaccharide.
In general, careful selection of the purification steps and analytical methodology can provide compositional data that is informative and relatable to physical attributes. In our laboratory this has provided the technology to quantify a wide range of natural materials, from sugar levels in human and animal feed, to novel cellulose derivatives for commercial coatings applications, the makeup of human milk oligosaccharides, through to providing patentable data on heparan sulfates as human therapeutics and even the composition of the preferred diet of the Kākāpō.
References
- Joseph, S. M.; Krishnamoorthy, S.; Paranthaman, R.; Moses, J.; Anandharamakrishnan, C. Carbohydrate Polymer Technologies and Applications 2021, 2, 100036.
- Thieker, D. F.; Hadden, J. A.; Schulten, K.; Woods, R. J. Glycobiology 2016, 26 (8), 786-787.
- Capila, I.; Linhardt, R. J. Angewandte Chemie International Edition 2002, 41 (3), 390-412.
- Turnbull, J. E. Science 2011, 334 (6055), 462-463.
- Jones, C. J.; Beni, S.; Limtiaco, J. F.; Langeslay, D. J.; Larive, C. K. Annual Review of Analytical Chemistry 2011, 4, 439-465.
- Sims, I. M.; Carnachan, S. M.; Bell, T. J.; Hinkley, S. F. Carbohydrate Polymers 2018, 188, 1-7.
- Wang, J.; Zhao, J.; Nie, S.; Xie, M.; Li, S. Food Chemistry 2023, 399, 133968.
- Song, Y.; Zhang, F.; Linhardt, R. J. Journal of Histochemistry & Cytochemistry 2021, 69 (2), 121-135.
- Chaplin, M. F. Reviews in Cell Biology and Molecular Medicine 2006.
- Speciale, I.; Notaro, A.; Garcia-Vello, P.; Di Lorenzo, F.; Armiento, S.; Molinaro, A.; Marchetti, R.; Silipo, A.; De Castro, C. Carbohydrate Polymers 2022, 277, 118885.
- Mäkelä, V.; Helminen, J.; Kilpeläinen, I.; Heikkinen, S. Journal of Magnetic Resonance 2016, 271, 34-39.
- A. Lewis personal communication to S.F.R. Hinkley (2023) "The response factor of a C-H correlation recorded in an HSQC is specific to that sugar and its exact substituents due to polarization transfer from adjacent nuclei. To this end a set of measurements of a standard with the exact molecular arrangement must be individually calibrated as these responses differ significantly." Callaghan Innovation, Gracefield, Lower Hutt.
- Chaplin, M.; Kennedy, J., Carbohydrate Analysis: A Practical Approach. 1994. ILR Press. ISBN: 9780199634491
- Menna, A.; Fischer-Stettler, M.; Pfister, B.; Andrés, G. S.; Holbrook-Smith, D.; Sánchez-Rodríguez, C. Bio-protocol 2020, 10 (5), e3546-e3546.
- Sims, I. M.; Newman, R. H. Carbohydrate Polymers 2006, 63 (3), 379-384.
- Kidgell, J. T.; Carnachan, S. M.; Magnusson, M.; Lawton, R. J.; Sims, I. M.; Hinkley, S. F. R.; de Nys, R.; Glasson, C. R. K. Carbohydrate Polymers 2021, 264, 118010.
- Saeman, J. F. Industrial & Engineering Chemistry 1945, 37 (1), 43-52.
- Carnachan, S. M.; Bootten, T. J.; Mishra, S.; Monro, J. A.; Sims, I. M. Food Chemistry 2012, 133 (1), 132-139.
- Popper, Z. A.; Bootten, T.; Harris, P.; Melton, L.; Newman, R., Chapter 13 in The Plant Cell Wall. Springer: 2011. ISBN 9781617790089.
- Quach, M. L.; Melton, L. D.; Harris, P. J.; Burdon, J. N.; Smith, B. G. Journal of the Science of Food and Agriculture 2001, 81 (3), 311-318.
- Ciucanu, I.; Kerek, F. Carbohydrate research 1984, 131 (2), 209-217.
- Harris, P. J.; Henry, R. J.; Blakeney, A. B.; Stone, B. A. Carbohydrate Research 1984, 127 (1), 59-73.
- Pettolino, F. A.; Walsh, C.; Fincher, G. B.; Bacic, A. Nature protocols 2012, 7 (9), 1590-1607.
- Kim, J.-B.; Carpita, N. C. Plant Physiology 1992, 98 (2), 646-653.
- Shea, E. M.; Gibeaut, D. M.; Carpita, N. C. Planta 1989, 179, 293-308.
- Stevenson, T. T.; Furneaux, R. H. Carbohydrate Research 1991, 210, 277-98.
- Kidgell, J.; Glasson, C. R.; Magnusson, M.; Sims, I. M.; Hinkley, S. F.; de Nys, R.; Carnachan, S. M., Ulvans are Not Equal-Linkage and Substitution Patterns in Ulvan Polysaccharides Differ with Ulva Morphology. Available at SSRN 4599341.
- Wardrop, D.; Keeling, D. British Journal of Haematology 2008, 141 (6), 757-763.
- Xu, D.; Esko, J. D. Annual review of biochemistry 2014, 83, 129-157.
- Sargison, L.; Smith, R. A.; Carnachan, S. M.; Daines, A. M.; Brackovic, A.; Kidgell, J. T.; Nurcombe, V.; Cool, S. M.; Sims, I. M.; Hinkley, S. F. Carbohydrate Polymers 2022, 282, 119081.
- Murali, S.; Rai, B.; Dombrowski, C.; Lee, J.; Lim, Z.; Bramono, D.; Ling, L.; Bell, T.; Hinkley, S.; Nathan, S. Biomaterials 2013, 34 (22), 5594-5605.
- Qiao, M.; Lin, L.; Xia, K.; Li, J.; Zhang, X.; Linhardt, R. J. Talanta 2020, 219, 121270.
- Shriver, Z.; Capila, I.; Venkataraman, G.; Sasisekharan, R. Heparin-A Century of Progress 2012, 159-176.
- Carnachan, S. M.; Hinkley, S. F. Bio-protocol 2017, 7 (7), e2197-e2197.
- Conrad, H. E., Heparin-binding proteins. Elsevier: 1997. ISBN 0121860604.
- Kozlowski, A. M.; Yates, E. A.; Roubroeks, J. P.; Tømmeraas, K.; Smith, A. M.; Morris, G. A. ACS Applied Materials & Interfaces 2021, 13 (4), 5551-5563.
- Pawar, N. J.; Wang, L.; Higo, T.; Bhattacharya, C.; Kancharla, P. K.; Zhang, F.; Baryal, K.; Huo, C. X.; Liu, J.; Linhardt, R. J. Angewandte Chemie 2019, 131 (51), 18750-18756.
- Nakajima, M.; Irimura, T.; Di Ferrante, N.; Nicolson, G. Journal of Biological Chemistry 1984, 259 (4), 2283-2290.
- Steel, R. A.; Nieman, T. A. Analytica Chimica Acta 1983, 155, 123-129.
- Trim, P. J.; Hopwood, J. J.; Snel, M. F. Analytical Chemistry 2015, 87 (18), 9243-9250.