Switchable solvents are liquids with properties that are rapidly and reversibly altered in response to specific changes in their environment. These solvents are promising alternatives to hazardous, volatile organic solvents used in chemical synthesis, plastic recycling and the separation of valuable components from complex agricultural waste. Cameron C. Weber investigates.
Introduction
Solvents are widely used for an array of chemical reactions, separations and purifications. Historically, volatile solvents have been preferred as they can be easily removed by distillation after the reaction or separation has been performed. However, there is increasing concern regarding the safety and environmental risks associated with the use of volatile solvents due to their flammability, the inhalation risk posed and their potential to contribute to atmospheric pollution. The emission of volatile solvents has also been proportionally increasing in comparison to the release of other volatile organic compounds. In the UK for example, solvents represented 35% of volatile organic compound emissions in 1990 but 63% in 2017, with the proportion still increasing (Fig. 1).1 Furthermore, many of the volatile solvents commonly used are toxic to both the environment and human health creating the need for the development of safe, environmentally benign, non-volatile solvents.2
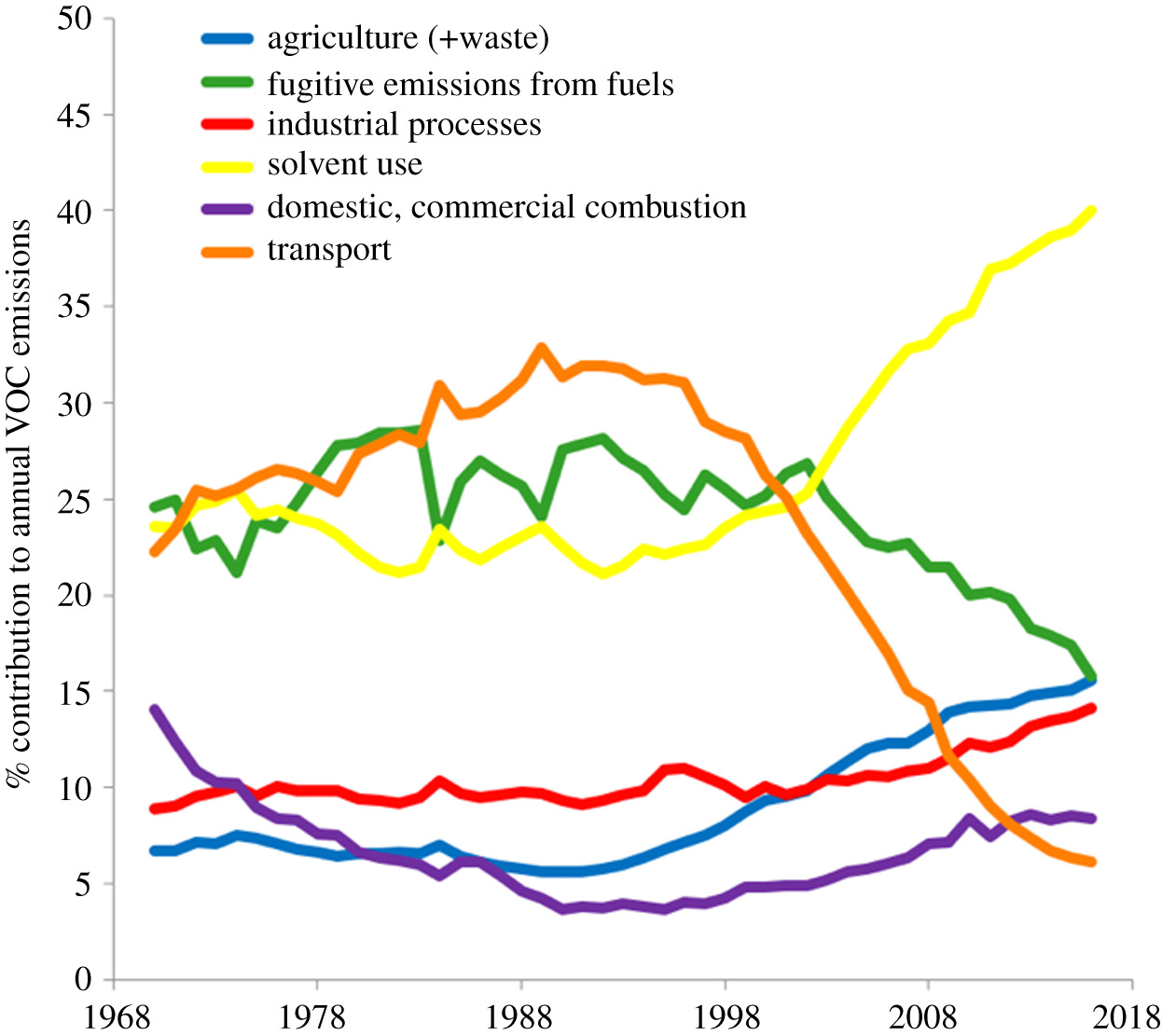
Fig. 1. Contribution to UK annual volatile organic compound emissions by source. Reproduced with permission from Lewis et al.1
Solvents are not incorporated into the final product of a chemical reaction and so represent an inherent waste product if they are not completely recovered and reused. Solvents often comprise around 85% by mass of the raw materials required to produce pharmaceuticals.3 While solvents can often be reused after distillation, some loss is inevitable, particularly for volatile solvents, leading either to uncontrolled loss into the environment or waste that is dealt with through energy-intensive incineration processes.
The safety and environmental risks posed by solvents is so pronounced that safer solvents are explicitly listed as one of the Twelve Principles of Green Chemistry in the foundational green chemistry book, “Green chemistry: theory and practice” written by Paul Anastas and John Warner.4 This is not the only principle relevant to solvent use, with more general principles such as the prevention of waste, safer chemical synthesis and inherently less hazardous chemistry being of relevance to reduced solvent use and the use of less harmful solvents.
Hence, it is clear that there is a need to eliminate solvents where possible and to develop safer, more efficient solvents for applications where they are required. Work in recent years has focused on solving the volatility issue, with the development of a multitude of non-volatile or less volatile alternative solvents such as ionic liquids and deep eutectic solvents.2 While these solvents address many of the safety and environmental challenges arising from volatile organic solvents, an enduring issue is the separation of solutes from these solvent systems. Unless the spontaneous separation of the solute can be achieved, e.g. by distilling a volatile solute or enabling its precipitation at a low temperature, separations usually require the use of an additional solvent to isolate the solute. This added solvent often needs to be volatile to facilitate the recovery of the solute and hence the overall process becomes reliant at least to some extent on volatile solvents. These challenges have meant that despite substantial research interest in non-volatile solvents under the umbrella of green chemistry, the scope of their implementation into industrial processes is often limited to cases where solutes are gases or highly volatile or can be chemically altered to facilitate recovery (e.g. the electrodeposition of metals).5- 6 The difficulty solving this separation issue has led to such non-volatile solvents being classified as ‘problematic’ rather than ‘recommended’ in the CHEM21 solvent selection guide, one of the most comprehensive guides evaluating the safety, human health risk and environmental risk of solvent use.7
The ideal “green” solvent is therefore subject to a series of seemingly conflicting demands. It needs to be non-volatile to reduce its flammability, potential to contribute to atmospheric pollution and risk to workers through inhalation. It also needs to be easily separable from solutes so that they can be recovered and the solvent can be recycled, without being dependent on the nature of the solute. Ideally this should all be accomplished under as close to ambient conditions as possible to help minimise energy use.
An innovative approach towards meeting all of these requirements simultaneously has been the development of switchable solvents. Switchable solvents undergo an abrupt change in their physicochemical properties on exposure to an external stimulus (i.e. the ‘switch’).8 By changing the nature of the solvent, it removes the need to physically remove the solvent by distillation as it can spontaneously separate from solutes or co-solvents, provided the change in properties is sufficient. This enables the use of non-volatile solvents while providing a more straightforward pathway to recover compounds dissolved in them.
Types of switchable solvents
Switchable solvents are usually classified by the nature of the switch used to generate the change in properties. Common methods used for switching include a change in temperature, change in pH or the addition or removal of CO2.8-10 The general mechanisms underpinning each of these classes of solvents are summarised in Figure 2.
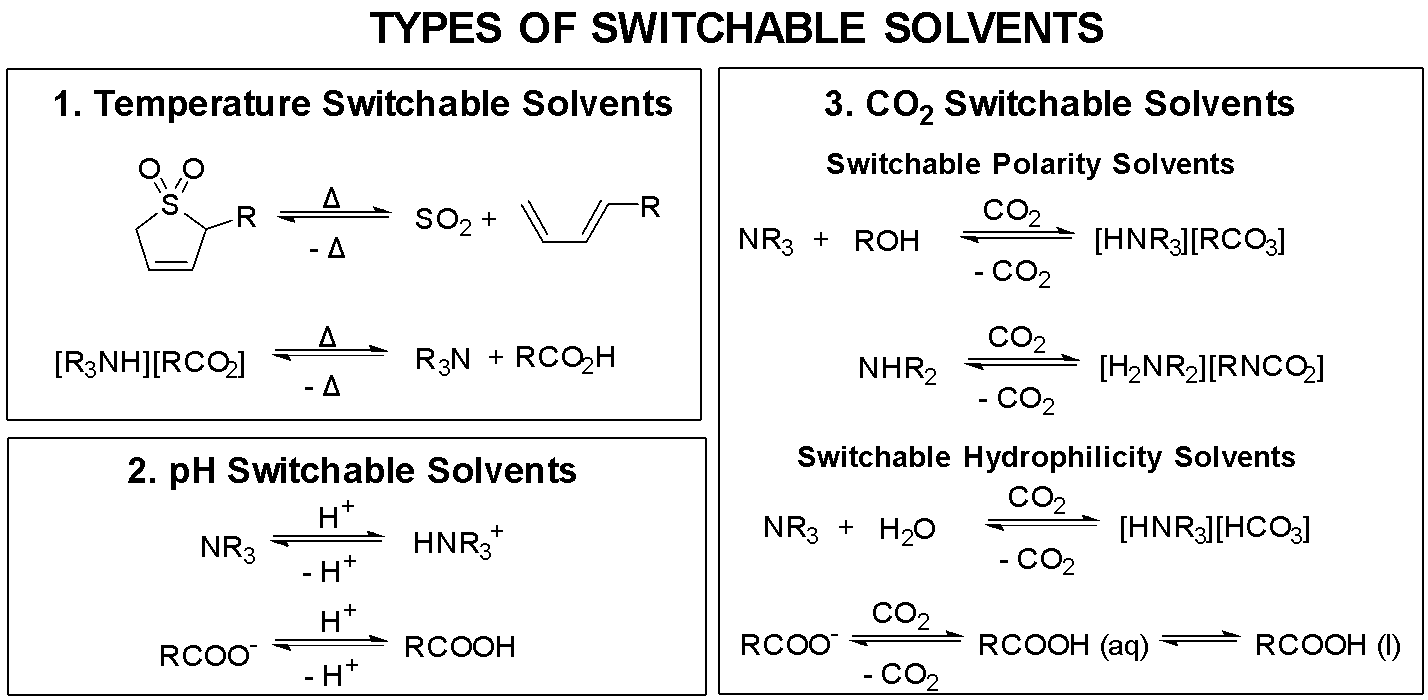
Fig. 2. Summary of the general reversible reactions underlying common types of switchable solvents. Box 1: Temperature switchable solvents; Box 2: pH switchable solvents; Box 3: CO2 switchable solvents.
Temperature as a switch
Two different mechanisms have been investigated for switchable solvents that undergo a change in response to temperature, also known as thermoswitchable solvents. The first includes those that reversibly degrade chemically, i.e. there is a temperature sensitive equilibrium with gaseous products.8 The second mechanism involves an abrupt change in miscibility with a co-solvent leading to spontaneous separation above or below a critical temperature.11-13
An example of a switchable solvent that undergoes a reversible decomposition equilibrium is piperylene sulfone (Fig. 2 Box 1: sulfone where R = CH3).8 Piperylene sulfone forms from the reaction of SO2 with trans-1,3-pentadiene, both gaseous reactants.14 On heating to temperatures above 100°C, piperylene sulfone spontaneously decomposes via a retro-cheletropic reaction into SO2 and trans-1,3-pentadiene which separate as gases, depositing any solutes that were dissolved. The piperylene sulfone can then be reformed in a separate vessel by cooling the gases to room temperature. Other related sulfones that can undergo similar reactions, such as butadiene sulfone, can also be used although the melting point of butadiene sulfone is 65°C (c.f. −12°C for piperylene sulfone) making it less practical as a solvent.15 A limitation of the use of these sulfones is the production of hazardous, toxic SO2 and diene gases.
The other approach to the use of temperature as a switch which has emerged over the past decade requires the use of a solution of the switchable solvent and a solvent with very high polarity, typically water.11-13 When the temperature is changed above or below the critical solution temperature, the phases spontaneously separate leading to the formation of a highly polar aqueous phase and a less polar organic phase. In one example, a 1:1 molar ratio of lidocaine and oleic acid were found to mix with water until they were heated above 30°C (Scheme 1),11 where a hydrophobic liquid phase containing lidocaine and oleic acid formed. Less than 0.35 wt% of lidocaine could be observed in the aqueous phase after separation, even when the initial lidocaine loading was greater than the mass of water present. Cooling the mixture to below 25°C led to the miscibility of the two phases, producing a single polar phase. This separation was attributed to changes in the degree of ionisation of the lidocaine and oleic acid with temperature, aiding the formation of a single phase at lower temperatures. Similar switchable behaviour has been seen from mixtures of alkanolamines and phenolics with water.12 While this method won’t lead to the spontaneous deposition of all solutes as could be observed for the gaseous switchable systems, the abrupt change in polarity can facilitate the precipitation of non-polar solutes from the hydrophobic phase when it mixes with water or the more efficient removal of organic-soluble components from aqueous waste streams due to the reversible miscibility of the extracting phase.

Scheme 1. Equilibrium between aqueous solution of lidocaine and oleic acid and biphasic water/organic mixture used as a switchable solvent system.
pH as a switch
pH switchable solvents rely on the protonation or deprotonation of the solvent to change its polarity, assisted by the presence of acidic or basic functional groups. For example, the addition of NaOH to a solution of N,N-dipropylammonium chloride has been used to facilitate the spontaneous phase separation of the N,N-dipropylamine from the aqueous solution.16 Conversely, the addition of base to hydrophobic carboxylic acids can facilitate their water miscibility.17 This phase change can then lead to the precipitation of organic compounds insoluble in polar aqueous salt solutions or else the more effective extraction of organic components from aqueous phases, as discussed previously for the thermoswitchable systems.
While the use of strong acids/bases can enable the efficient and selective switching of the polarity of these substances, it generates stoichiometric quantities of salt waste. These salts can be benign (e.g. NaCl in the previous example); however, they will accumulate through successive cycles and can limit the recyclability of the system. This indicates the need for a ‘traceless’ switching system which does not lead to cumulative waste generation within the solution.
CO2 as a switch
CO2 was the first ‘switch’ used for application with switchable solvents18 and has the advantage of being abundant, inexpensive, non-toxic and simple to remove, averting the accumulation of salts previously highlighted for pH-switchable solvents.
The first CO2-switchable system reported was a 1:1 molar ratio mixture of 1,8-diazabicyclo-[5.4.0]-undec-7-ene (DBU) and hexan-1-ol.18 The addition of CO2 at atmospheric pressure led to the formation of an ionic liquid based on the protonation of the DBU and generation of a hexylcarbonate anion from the hexan-1-ol (Scheme 2). The reaction could be reversed by purging the solution with N2 gas at atmospheric pressure to remove the CO2. CO2-switchable solvents using this concept are known as switchable polarity solvents and include the use of primary amines in place of the alcohol to form carbamate salts or the use of secondary amines which do not require any additional components to form an ammonium carbamate salt.
A limitation of this initial approach was its water sensitivity. The HCO3− salt of DBU and of alternative amines or amidines are solids and form on exposure of the amine or amidine to water and CO2. This salt then crystallises and prevents the reversible formation of the switchable solvent.

Scheme 2. CO2-switchable solvent based on reversible reaction of CO2 with DBU and hexan-1-ol.
Switchable hydrophilicity solvents (SHSs) were developed in 2010 to address the water sensitivity of the first generation of CO2-switchable solvents.19-20 SHSs use a hydrophobic amine or amidine that reacts reversibly with CO2 and water to form a concentrated aqueous solution of the HCO3− salt (Fig. 2, Box 3). Purging this solution with nitrogen, combined with gentle heating (e.g. at 50°C) leads to the regeneration of the hydrophobic amine or amidine which then phase separates from the water. By embracing the use of water, SHSs bypass the need for anhydrous systems and the rigorous drying of solutes or matrices.
A major restriction in the design of SHSs is the need to balance the hydrophilicity and basicity of the amine or amidine. If it is too hydrophobic then the polar switched form will not mix with water and if it is too hydrophilic then the less polar form will mix with water. Similarly, the amine/amidine needs to be sufficiently basic to react with CO2 but not too basic or the reaction is no longer reversible. Jessop and coworkers identified that the ideal range for a SHS is an amine or amidine with a log Kow (octanol-water partition coefficient) value of 1.2-2.5 and log pKa of the conjugate acid between 9.5–11 to ensure these conditions are met.21
While these ranges provide scope for the inclusion of a variety of different amines and amidines, an ongoing issue has been the inherent toxicity of many of the compounds that satisfy these conditions.17 Recently, SHSs that don’t require an amine have been reported. These feature a hydrophobic carboxylic acid.17 This acid is partially deprotonated by the substoichiometric addition of a base, such as NaOH, to enable its miscibility with water. The acid can then be recovered from the aqueous solution by the addition of CO2 to reform the hydrophobic acid (Fig. 2, Box 3). While the pKa of most carboxylic acids would suggest that they shouldn’t react completely with CO2, the separation of the water immiscible carboxylic acid drives the equilibrium towards its formation although the complete phase separation of these acids remains a challenge.
Applications of switchable solvents
Since their inception less than 20 years ago, switchable solvents have been explored for a variety of different applications including chemical synthesis, analysis of complex mixtures, fractionation of biomass sources and plastic recycling. The switchable concept, including CO2-switchable systems as well as those responsive to light, pH and redox chemistry, has also been embedded in materials to develop switchable surfactants, catalysts, particles and gels although for brevity these applications will not be discussed here.9 Some selected examples of switchable solvents are highlighted below, emphasising those that take advantage of the ease of separation from the solvent.
Chemical synthesis
Switchable solvents have been investigated for applications in chemical synthesis with the aim of simplifying the separation of reaction products and the recycling and regeneration of the solvent and catalysts. Examples below will focus on those where the switchable solvent was used as the reaction solvent itself rather than as part of the reaction work-up, although there have been successful examples of the latter.22
Piperylene sulfone was studied as a more easily recoverable alternative to DMSO as a solvent for substitution reactions involving benzyl chloride.23 While reaction rates for the benzyl chloride substitution in piperylene sulfone were typically slower than in DMSO, it offered the advantage of being able to easily recover the reaction products by decomposing, removing and regenerating the solvent (Fig. 3).

Fig. 3. Process for the use of piperylene sulfone as a reaction solvent, including its recovery. Reproduced from Vinci et al.23 with permission from the Royal Society of Chemistry.
CO2-switchable polarity solvents involving amidine/alcohol solvents have been investigated for a range of chemical reactions including Claisen-Schmidt condensations, Heck coupling and the cyanosilylation of cyclohexanone among others.24 While the Heck coupling could be performed efficiently, challenges were observed for some of the other reactions explored. For the condensation reactions (Scheme 3), the amidine acted as a basic catalyst and solvent enabling the efficient formation of the enone products although the water formed as a co-product of the condensation caused the generation of the amidine HCO3− salt which inhibited solvent recycling. The addition of magnesium sulfate as a drying agent reduced the formation of this undesired byproduct, facilitating solvent recycling with no loss in activity observed over 3 cycles. The cyanosilylation reaction was similarly affected by an undesirable side reaction. In this case, a side reaction between the solvent and trimethylsilyl cyanide (the cyanosilylation reagent) led to a build-up of the cyanosilylated amidine over time, reducing its efficacy as a solvent and substantially decreasing the observed product yield upon recycling. These examples highlight that the solvent reactivity, a requirement for the CO2-switchable behaviour, can lead to challenges in terms of cross-reactivity when these solvents are used in chemical synthesis.
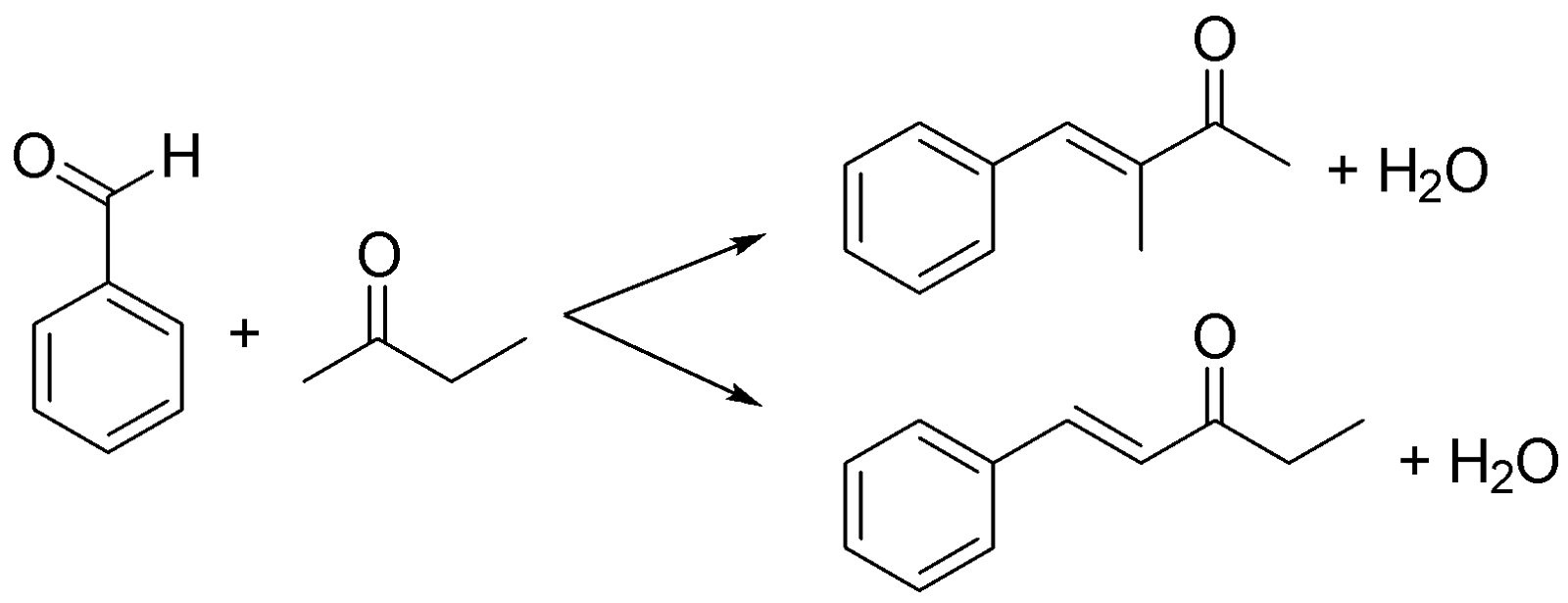
Scheme 3. Claisen-Schmidt condensation studied in switchable polarity solvents by Hart et al.24
One area of chemical synthesis where CO2-switchable solvents have had significant recent success has been cellulose solubilisation and derivatisation, with the solvent critical for enabling both aspects.25-26 It has been found that a solvent system comprising DBU and DMSO can solubilise cellulose upon addition of CO2. Cellulose solubility is driven by the conversion of the alcohol groups on the cellulose to carbonates.26 After solubilisation, cellulose was found to react with isothiocyanates to form thiocarbamates with controllable degrees of substitution, with the DBU acting as a catalyst for this transformation in addition to its role as a solvent (Scheme 4). The derivatised cellulose could then be recovered by the removal of CO2 and the solvent components could be recovered in 79-96% efficiency.
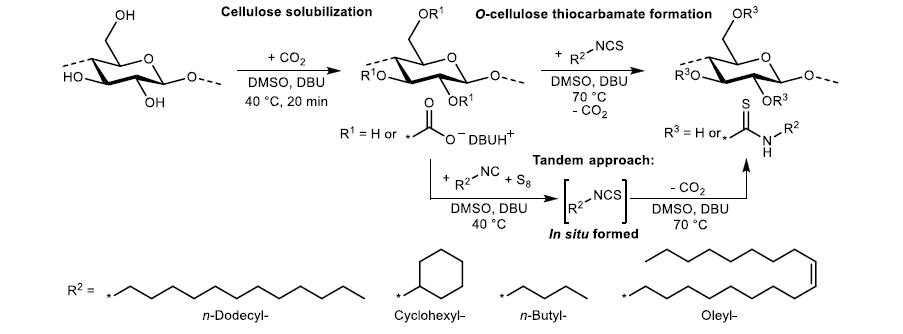
Scheme 4. Solubilisation of cellulose using a DBU/DMSO CO2-switchable solvent system. Reprinted with permission from Wolfs et al.25 Copyright 2021 American Chemical Society.
From the above examples, it is clear that the implementation of switchable solvents to chemical synthesis requires careful consideration of the inherent solvent reactivity. While poor solvent selection can lead to undesirable cross-reactivity, appropriate selection can generate multifunctional solvents that catalyse the desired reaction and provide the straightforward separation of reaction products.
Separations and purifications
Separations, extractions and purifications are the most highly studied application of switchable solvents. Central to many of these applications is the ability for the desired solute to spontaneously separate after switching the solvent. SHSs in particular are incredibly valuable for the fractionation of complex mixtures for two key reasons. Firstly, they can perform extractions and separations using both non-polar and polar forms of the solvent. This essentially combines the regeneration of the solvent with further extraction steps. SHSs are also resilient to the presence of water in the matrix, avoiding the energetic cost associated with drying substrates. These energy savings are particularly important when using biomass sources which can contain substantial quantities of water.
A circular isolation strategy using SHSs has been implemented in the fractionation of algae with the aim of separating lipids for biodiesel production alongside carbohydrates as potential platform chemical feedstocks. 27-28 The optimal solvent explored for these extractions was N,N-dimethylcyclohexylamine (DMCHA) which could extract nearly 50% of the protein and carbohydrates from the algae in its hydrophilic form (i.e. the form generated after adding water and CO2), followed by 93% of lipids when the algal residue was further extracted by DMCHA in its hydrophobic form. Each of these fractions could be easily isolated when the solvent was switched. For the proteins and carbohydrates this was in the form of an aqueous phase that separated spontaneously from the hydrophobic DMCHA and for the lipids an oily phase was formed when CO2 and water were added. This process provided proof of principle for the circularity of switchable solvent extractions, where every form of the solvent could be used to aid the separation of a complex mixture.
Our group, in conjunction with researchers at Scion, has recently demonstrated the use of SHSs for the fractionation of Pinus radiata bark.29 60 million dry tonnes of bark waste is produced per year from the forestry industry and despite its potential as a source of biorenewable chemicals, it is largely used for low value applications such as garden mulch or boiler fuel. We discovered that the switchable solvent DMCHA and its secondary amine counterpart N-methylcyclohexylamine (MCHA) were the most effective of a range of SHSs explored for the extraction of bark, with other solvents not yielding isolable products. Extractions using the hydrophobic form of the solvents led to the selective recovery of fatty acids and alcohols which could be separated by filtration after the addition of CO2 and water. Further extraction of the bark residue by the hydrophilic form yielded exclusively bark tannins when DMCHA was used as a solvent and a combination of small amounts of lignin, carbohydrates and tannins were obtained from MCHA. DMCHA could be successfully recycled with no loss of efficiency and without significantly extracting core structural components of the bark. This highlights how switchable solvent technology may have potential to help fractionate complex bio-based mixtures into individual constituents for further applications.
The efficacy of switchable solvent extraction has been combined with the inherent reactivity of these solvents for the simultaneous extraction of triglycerides from spent coffee ground waste and their conversion into biodiesel.30 In this process, DBU was used as both a component of the solvent and basic catalyst. Under optimal conditions, DBU and methanol were combined with the spent coffee ground waste at 130°C for 60 mins and a biodiesel yield of 96% could be obtained. The biodiesel could be separated from the solvent by the addition of CO2 and the solvent then regenerated by heating under a flow of nitrogen gas. The solvent could be reused for up to 10 cycles with no loss in reactivity observed.
Plastic recycling
Plastic waste is a major global challenge due to both the scale of production (> 450 million tonnes per year)31 and the poor biodegradability of many plastic products. Furthermore, the majority of plastics are produced from petrochemical sources, providing a further environmental imperative to reduce their production. One approach is to recycle existing plastics to reduce the need for further production, although successful recycling is complicated by the use of complex mixtures in many packaging applications and the energy requirements associated with some recycling processes.
Expanded polystyrene is often used as an insulator and packing material, but is difficult to efficiently recycle due to its relatively low density and high air content (90-95 vol%) which leads to high transport costs caused by the large material volume relative to the mass of plastic.17 Both amine and carboxylic acid SHSs have been investigated for the recycling of this foam.17, 20 For both amine and carboxylic acids, the hydrophobic form dissolves the polystyrene leading to the release of the trapped air. The addition of CO2 and water leads to the precipitation of the polystyrene from the solvent. The reconstituted polystyrene can be extruded and repurposed without any significant loss of mechanical properties. A limitation of the use of SHSs for this application is the incorporation of small quantities of residual solvent in the resultant polystyrene, particularly problematic for the odorous and toxic amine-based solvents. While the use of less harmful carboxylic acids reduces the impact of residual solvent inclusion, further optimisation is required for it to become a viable recycling option.
The recycling of multilayered packaging materials has been investigated using SHSs, leveraging their potential to facilitate the separation of complex mixtures.32-33 In an initial study, Samori et al.33 examined the recycling of multilayered aseptic food packaging which comprised paper, aluminium and low density polyethylene (LDPE) layers (Fig. 4). While the paper layers can be easily separated through a hydropulping process, the remaining aluminium and LDPE are difficult to separate. Alternative approaches include the use of undesirable solvents such as xylenes or chloroform for the isolation of both phases or the use of thermal conditions such as plasma or incineration which only allows for energy recovery from the LDPE and affects the quality of the recovered aluminium due to char formation and oxidation. DMCHA was explored as the switchable solvent for this separation. In the first step, the LDPE was solubilised in the non-polar DMCHA, leaving the aluminium residue which was filtered and then washed with carbonated water to remove traces of DMCHA. The addition of water and CO2 to the LDPE and DMCHA solution led to the precipitation of the LDPE which was filtered and washed with water. The DMCHA could then be recovered and reused by gentle heating at 40°C, leading to its separation from the water. > 99% of the aluminium and 93% of the LDPE could be recovered by this approach. Life cycle assessment indicated that this approach led to at least a 12.5% reduction in greenhouse gas emissions compared to the next best industrial process.
This approach was then applied to more complex food product packaging that contained mixed plastic layers.32 As the waste material was more complex, the separation was facilitated by sonication (i.e. the use of ultrasound) which enabled the near quantitative recovery of the switchable solvent as well as the aluminium and polymer layers.

Fig. 4. Recycling of LDPE and aluminium from aseptic food packaging. Reproduced from Samori et al.33 with permission from the Royal Society of Chemistry.
Conclusions
Switchable solvents represent a potential solution to the enduring challenge of developing environmentally benign, non-volatile solvents that can be easily separated from solutes or co-solvents. Considerable advances have been made over the past two decades including the deployment of switchable solvents for chemical synthesis, separations and purifications as well as for plastic recycling.
Nonetheless, further work is still required to identify genuinely benign components for these solvents, avoiding the use of toxic amines and petrochemical derived components. There is also the need to ensure the absolute separation of the solvent from solutes or other solvent phases to avoid contamination.
Finally, the application of these solvents in chemical synthesis will require careful consideration of the cross-reactivity of the solvent with potential substrates but if chosen correctly can yield more efficient reactions. Numerous future directions emerge for these innovative solvent systems with scope for extension into new fields, such as the use of light or redox-activated switching or the combination of orthogonal switchable groups to facilitate more nuanced separations. As our understanding of these solvents grows, so too will their use in wider and more diverse applications.
References
- Lewis, A.C.; Hopkins, J.R.; Carslaw, D.C.; Hamilton, J.F.; Nelson, B.S.; Stewart, G.; Dernie, J.; Passant, N.; Murrells, T. Philosophical Transactions of the Royal Society A: Mathematical, Physical and Engineering Sciences, 2020, 378, 20190328.
- Clarke, C.J.; Tu, W.-C.; Levers, O.; Bröhl, A.; Hallett, J.P. Chemical Reviews, 2018, 118, 747-800.
- Sheldon, R.A. Green Chemistry, 2005, 7, 267-278.
- Anastas, P.T.; Warner, J.C., Green chemistry: theory and practice, Oxford University Press, Oxford, 1998.
- Smith, E.L.; Abbott, A.P.; Ryder, K.S. Chemical Reviews, 2014, 114, 11060-11082.
- Greer, A.J.; Jacquemin, J.; Hardacre, C. Molecules, 2020, 25, 5207.
- Prat, D.; Wells, A.; Hayler, J.; Sneddon, H.; McElroy, C.R.; Abou-Shehada, S.; Dunn, P.J. Green Chemistry, 2016, 18, 288-296.
- Pollet, P.; Eckert, C.A.; Liotta, C.L. Chemical Science, 2011, 2, 609-614.
- Jessop, P.G.; Mercer, S.M.; Heldebrant, D.J. Energy & Environmental Science, 2012, 5, 7240-7253.
- Jessop, P.G. Aldrichimica Acta, 2015, 48, 18-21.
- Longeras, O.; Gautier, A.; Ballerat-Busserolles, K.; Andanson, J.-M. ACS Sustainable Chemistry & Engineering, 2020, 8, 12516-12520.
- Xiong, D.; Zhang, Q.; Ma, W.; Wang, Y.; Wan, W.; Shi, Y.; Wang, J. Separation and Purification Technology, 2021, 265, 118479.
- Castaneda Corzo, J.; Ballerat-Busserolles, K.; Coxam, J.-Y.; Gautier, A.; Andanson, J.-M. Journal of Molecular Liquids, 2023, 377, 121468.
- marus, G.A.; Vyhmeister, E.; Pollet, P.; Donaldson, M.E.; Llopis-Mestre, V.; Faltermeier, S.; Roesel, R.; Tribo, M.; Gelbaum, L.; Liotta, C.L.; Eckert, C.A. Industrial & Engineering Chemistry Research, 2011, 50, 23-27.
- Huang, Y.; Ureña-Benavides, E.E.; Boigny, A.J.; Campbell, Z.S.; Mohammed, F.S.; Fisk, J.S.; Holden, B.; Eckert, C.A.; Pollet, P.; Liotta, C.L. Sustainable Chemical Processes, 2015, 3, 13.
- Shahvandi, S.K.; Banitaba, M.H.; Ahmar, H. Talanta, 2018, 184, 103-108.
- Cunha, I.T.; McKeeman, M.; Ramezani, M.; Hayashi-Mehedy, K.; Lloyd-Smith, A.; Bravi, M. Jessop, P.G. Green Chemistry, 2022, 24, 3704-3716.
- Jessop, P.G.; Heldebrant, D.J.; Li, X.; Eckert, C.A.; Liotta, C.L. Nature, 2005, 436, 1102-1102.
- Jessop, P.G.; Phan, L.; Carrier, A.; Robinson, S.; Dürr, C.J.; Harjani, J.R. Green Chemistry, 2010, 12, 809-814.
- Jessop, P.G.; Kozycz, L.; Rahami, Z.G.; Schoenmakers, D.; Boyd, A.R.; Wechsler, D.; Holland, A.M. Green Chemistry, 2011, 13, 619-623.
- Vanderveen, J.R.; Durelle, J.; Jessop, P.G. Green Chemistry, 2014, 16, 1187-1197.
- Phan, L.; Andreatta, J.R.; Horvey, L.K.; Edie, C.F.; Luco, A.-L.; Mirchandani, A.; Darensbourg, D.J.; Jessop, P.G. The Journal of Organic Chemistry, 2008, 73, 127-132.
- Vinci, D.; Donaldson, M.; Hallett, J.P.; John, E.A.; Pollet, P.; Thomas, C.A.; Grilly, J.D.; Jessop, P.G.; Liotta, C.L.; Eckert, C.A. Chemical Communications, 2007, DOI: 10.1039/B616806J, 1427-1429.
- Hart, R.; Pollet, P.; Hahne, D.J.; John, E.; Llopis-Mestre, V.; Blasucci, V.; Huttenhower, H.; Leitner, W.; Eckert, C.A.; Liotta, C.L. Tetrahedron, 2010, 66, 1082-1090.
- Wolfs, J.; Nickisch, R.; Wanner, L.; Meier, M.A.R. Journal of the American Chemical Society, 2021, 143, 18693-18702.
- Onwukamike, K.N.; Tassaing, T.; Grelier, S.; Grau, E.; Cramail, H.; Meier, M.A.R. ACS Sustainable Chemistry & Engineering, 2018, 6, 1496-1503.
- Boyd, A.R.; Champagne, P.; McGinn, P.J.; MacDougall, K.M.; Melanson, J.E.; Jessop, P.G. Bioresource Technology, 2012, 118, 628-632.
- Cicci, A.; Sed, G.; Jessop, P.G.; Bravi, M. Green Chemistry, 2018, 20, 3908-3911.
- Patel, S.D.; Brunschwig, C.; Robertson, M.; Murray, R.; Thumm, A.; Raymond, L.; Hill, S.J.; Weber, C.C. Industrial Crops and Products, 2024, 209, 117966.
- Nguyen, H.C.; Nguyen, M.L.; Wang, F.-M.; Liang, S.-H.; Bui, T.L.; Ha, H.H.; Su, C.-H. Bioresource Technology, 2019, 289, 121770.
- Ritchie, H.; Samborska, V.; Roser, M. OurWorldInData.org, 2023 (accessed 16/02/2024).
- Mumladze, T.; Yousef, S.; Tatariants, M.; Kriūkienė, R.; Makarevicius, V.; Lukošiūtė, S.-I.; Bendikiene, R.; Denafas, G. Green Chemistry, 2018, 20, 3604-3618.
- Samorì, C.; Cespi, D.; Blair, P.; Galletti, P.; Malferrari, D.; Passarini, F.; Vassura, I.; Tagliavini, E. Green Chemistry, 2017, 19, 1714-1720.