The rapid expansion of visible light-driven chemistry in the 21st century has relied upon precious metal photosensitisers. Now, earth-abundant alternatives for sustainable photochemistry have a bright and exciting future. Conor J. Doran, Sian E. Dillon and Christopher B. Larsen investigate.
Introduction
The use of photosensitisers to harness the energy inherent in visible light has opened the doors to a new realm of synthetic chemistry and helped secure the photon’s role as a 21st century reagent.
Most organic compounds either do not absorb visible light or do so very weakly. Photosensitisers are molecules that can absorb visible light, accessing excited states that are sufficiently long-lived to undergo bimolecular electron-/energy transfer reactions with organic substrates.1–3 This process is usually catalytic in nature, with the excited state photosensitiser subsequently returning back to the ground state (albeit often through the use of a sacrificial redox agent), ready to absorb another incident photon (packet of light energy). Coordinatively saturated octahedral transition metal complexes have played a pivotal role in the development of applications that leverage this excited state reactivity, such as photoredox catalysis, energy-transfer catalysis and photon upconversion, and remain among the most popular photosensitisers to date. Especially popular are precious metal complexes with the d6 electronic configuration, particularly RuII polypyridyl complexes and cyclometalated IrIII complexes.1
Upon visible light excitation, these precious metal complexes quickly and efficiently access long-lived 3MLCT (metal-to-ligand charge-transfer) excited states that are primed to undergo bimolecular electron-/energy transfer reactions.1 These long-lived excited states, with properties drastically different to that of the ground state complex, are well-suited for applications in energy technologies such as dye-sensitised solar cells, and synthetic chemistry as efficient photoredox/energy-transfer catalysts.2–4
The exponentially rising popularity of visible light-mediated chemistry, combined with the cost and scarcity of these precious metals, has recently prompted research into their replacement with more sustainable and less expensive earth-abundant elements, with particular focus on first-row transition metal complexes.5–7 Fig. 1 illustrates the relative abundance of the elements in the Earth’s crust.8
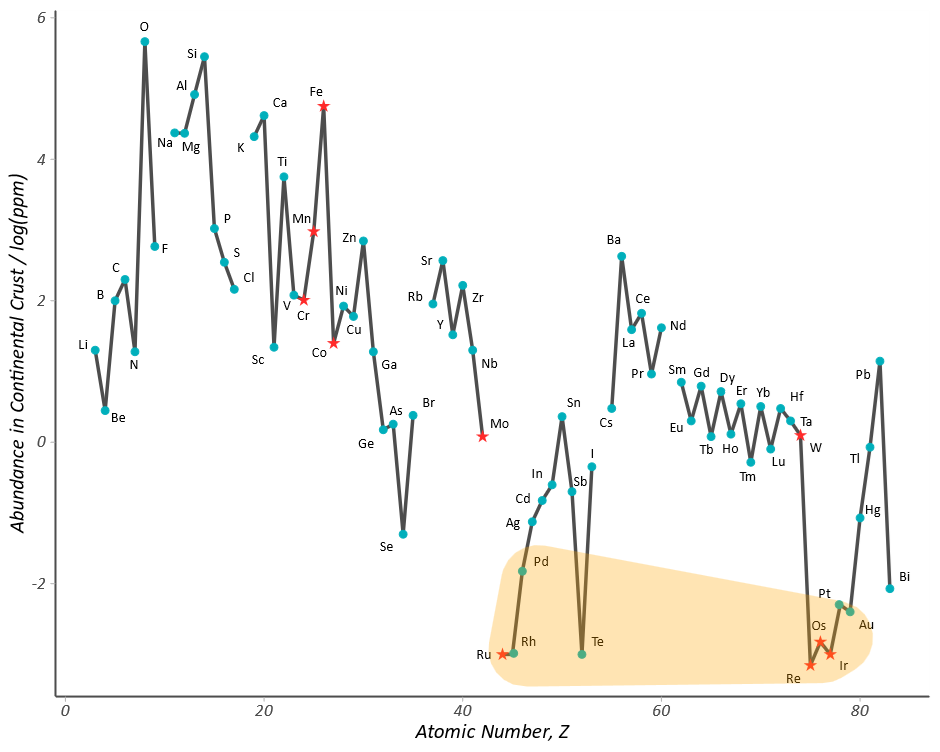
Fig. 1. Abundance of the elements in Earth’s crust.8 Elements relevant to d6 complexes are indicated (★), and precious metals highlighted in orange.
Several innovative design approaches now exist, including targeting LMCT (ligand-to-metal charge-transfer) excited states in complexes of metals with empty d orbitals,9,10 and MLCT states in complexes of metals with full d orbitals,11 as well as the emerging field of organic photosensitisers.12 This overview will focus on attempts to mimic the favourable photophysical properties of octahedral d6 precious metal (e.g. RuII, IrIII) complexes in isostructural and isoelectronic earth-abundant analogues.13 Specifically, we will focus on the development of FeII and CoIII complexes bearing polypyridyl and carbene-based ligands, as well as isocyanide complexes of W0, Mo0, Cr0 and MnI.
Photophysics
The underlying challenge associated with replacing second and third row metals with isoelectronic first-row metals is the inherent differences in their electronic structure arising from the primogenic effect – first-row metals possess contracted d orbitals relative to their heavier counterparts due to a lack of radial nodes in their wavefunctions.14 This weakens metal-ligand bonding interactions, decreasing the ligand-field strength and thereby stabilising ligand-field states, which provide an efficient pernicious pathway to depopulate the photochemically active MLCT state. As such, retention of the desired photoreactivity when moving to the first-row transition metals is markedly complex. This is best exemplified by comparing [Ru(bpy)3]2+ and [Fe(bpy)3]2+: both complexes exhibit similar electronic absorption spectra, but the former has a 3MLCT state lifetime of ~1 μs, whereas the latter has its excited state deactivate within ~50 fs to the ligand-field manifold, terminating in a low energy, photochemically inactive 5T2g state with a lifetime of 650 ps (Fig. 2).13 This limitation significantly restricts the practical use of FeII polypyridyl complexes.
Ligand design has therefore played an important role in accessing photochemical reactivity in octahedral earth-abundant transition metal complexes with the d6 electronic configuration, as will be discussed in the following sections.
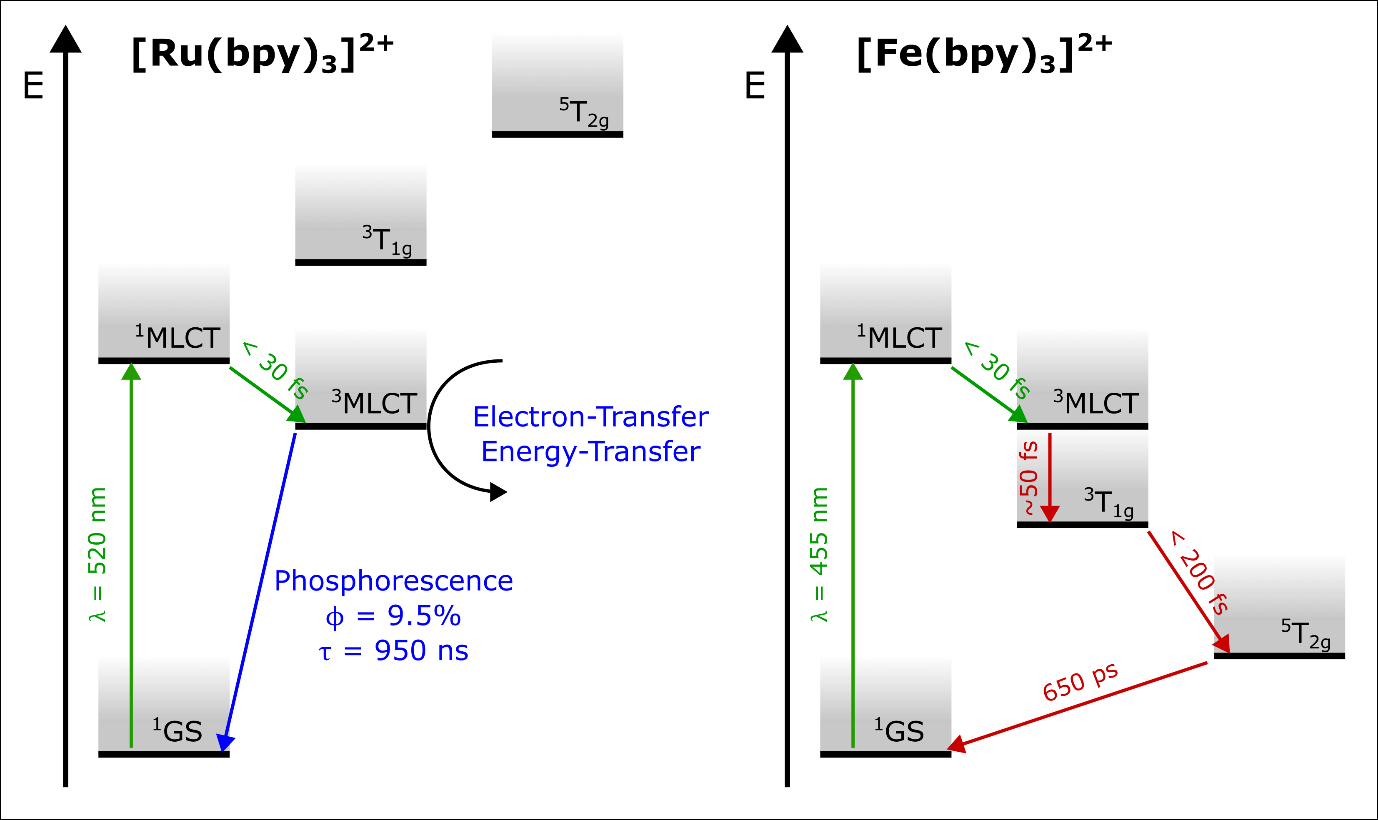
Fig. 2. Simplified energy level diagrams of [Ru(bpy)3]2+ and [Fe(bpy)3]2+, highlighting relevant excited-state deactivation pathways.
Iron(II)
Photoactive complexes of iron have long represented a key aspiration of the photochemistry community—iron being the fourth most abundant element in the earth’s crust, behind only oxygen, silicon and aluminium.8,15 It has, at varying points, been described as the “holy grail” of inorganic photocatalysis.15 Isoelectronic with RuII, FeII appears the obvious replacement.15,16
Although there have been decades of research into the photophysical properties of FeII complexes, it is only in the last few years that significant progress has been achieved towards the goal of developing FeII photosensitisers.15,17 In 2018, the Wärnmark group explored the use of mesoionic carbenes as strong σ-donor ligands to maximise the ligand-field in FeII complexes (Fig. 3a).18,19 The resulting complex, 1, possesses a 3MLCT excited state with a 528 ps lifetime – an order of magnitude longer than any previously existing FeII complex.18 However, the mesoionic carbenes increase the electron density at the metal centre to such an extent that 1 spontaneously oxidises in air to its FeIII congener. Serendipitously, this FeIII congener of 1 luminesces from a 2LMCT state with a 100 ps lifetime, representing the first example of charge-transfer luminescence from an iron complex.18 More recent ligand designs have pushed the 2LMCT state lifetime into the nanosecond regime, which has opened their potential as photoredox catalysts.15,17,19–21
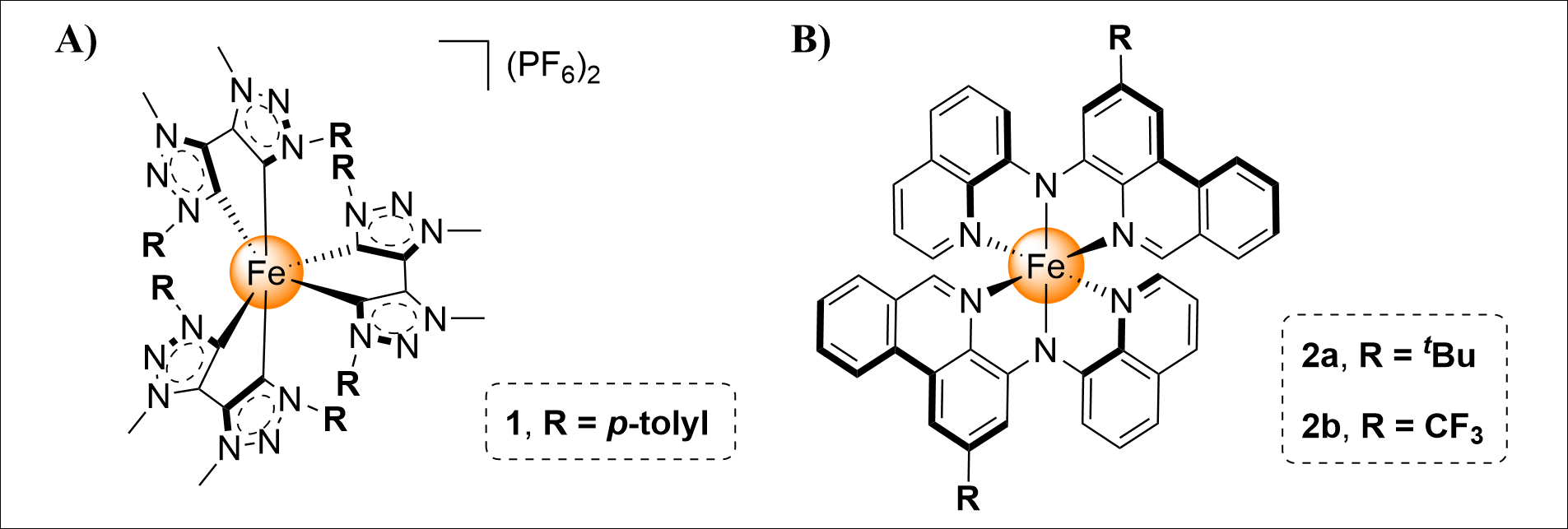
Fig. 3. (A) Wärnmark’s FeII mesoionic carbene complex. (B) Herbert’s FeII diarylamido complexes.
In 2019, the Herbert group reported a conceptually different approach, employing weak-field amido donor ligands, resulting in complexes 2a-b with panchromatic absorption and nanosecond CT excited state lifetimes (Fig. 3b).21 By employing weak-field amido ligands, mixing of the metal t2g with filled ligand-based orbitals, N(2p), results in the highest occupied molecular orbital (HOMO) being an antibonding orbital with mixed metal-ligand character. The consequent πanti-bonding-to-ligand charge transfer state (PALCT) excited states are substantially stabilised compared to the MLCT excited states of similar FeII polypyridyl complexes, whereas the enhanced metal-ligand covalency minimises the stabilisation of the ligand-field states arising from the weaker ligand field due to reduced interelectronic repulsion as a consequence of the nephelauxetic effect.21,22 The measured lifetimes are > 2 ns for both complexes, at that stage, an order of magnitude greater than any other published FeII complex.21 This was attributed to excited-state population transfer from the 3PALCT state to the ligand-field manifold having a markedly higher activation barrier than from the 3MLCT states of traditional FeII polypyridyl complexes.21–24
In 2022, the Berkefeld group, inspired by a computational prediction by the Dixon group,25,26 developed a cyclometalated FeII complex, 3, that exhibits near-infrared 3MLCT luminescence, representing the first example of MLCT luminescence in an FeII complex (Fig. 4a).27 Complex 3 features both bipyridine-N and phenyl-C donors that contribute to the 3MLCT being the lowest energy excited state.27 Redox studies suggested the suitability of complex 3 for aryl radical formation reactions analogous to traditional photosensitisers. Experimentally, radical cross-coupling of 4-chlorobromobenzene and benzene was observed (Fig. 4b).27
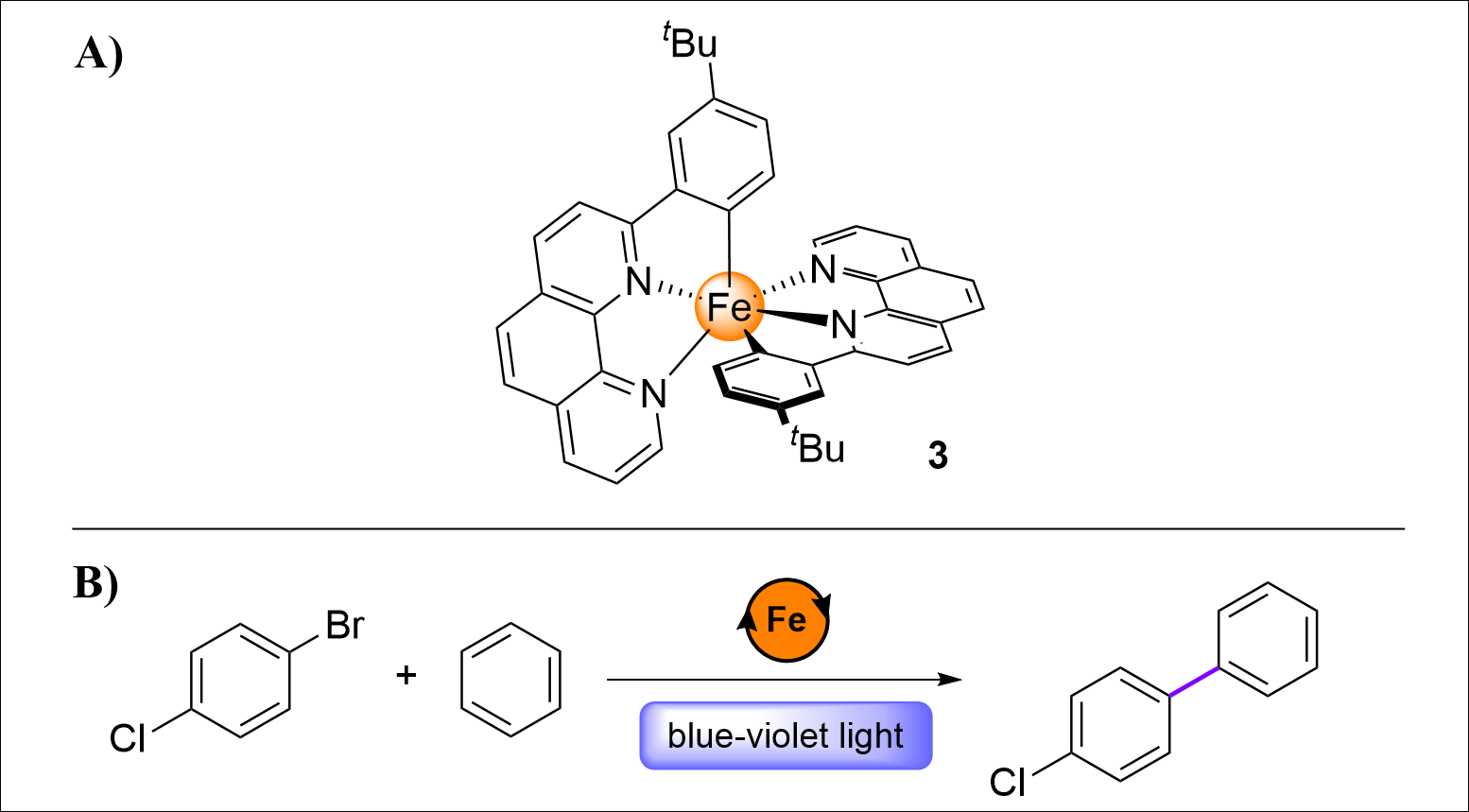
Fig. 4. (A) Berkefeld’s cyclometallated FeII complex. (B) Light-driven radical cross-coupling of 4-chlorobromobenzene and benzene by 3.
Cobalt(III)
Although cobalt is ~2,000 times less abundant than iron in the earth’s crust, it is still more than 10,000 times more abundant than ruthenium.8 Given cobalt’s relative abundance in the earth’s crust, and CoIII inherently possessing a stronger ligand field than FeII,28 it represents an attractive target for earth-abundant photosensitisers.17,29 In addition, the preferential accessibility of the CoII oxidation state relative to CoIV make CoIII complexes promising candidates for photooxidants.17 However, it should be noted that the role of cobalt in modern battery technology has put a large demand on cobalt mining, an extractive and exploitative process that has growing geopolitical and ethical implications.30
The inaccessibility of the CoIV oxidation state has consequences on the optical and photophysical properties of CoIII complexes relative to FeII — MLCT excited states are significantly raised in energy, such that they no longer absorb in the visible spectrum (CoIII polypyridyl complexes are typically yellow in colour).28,29 Although the ligand-field states are destabilised relative to those of homologous FeII complexes, the 3T1g state remains reasonably low in energy (< 15,000 cm-1), with excited state lifetimes on the order of a few nanoseconds.28 The McCusker group have recently demonstrated that the 3T1g state of CoIII polypyridyl complexes obeys the famous ‘Energy Gap Law’, unlike the 5T2g state of homologous FeII complexes.28,29,31 The important consequence of this finding is that as the excited state energy is raised (enhancing photochemical reactivity) its lifetime correspondingly increases, enhancing the probability of bimolecular reactions.28,29 Indeed, a collaboration between the McCusker and MacMillan groups has recently demonstrated that the 3T1g state of complex 4 can be utilised for photoredox catalysis, particularly in the (industrially useful) formation of C(sp2)-N bonds (Fig. 5a).31 The groups report efficient coupling of aryl amides with aryl boronic acids (Fig. 5b). The developed method provides promising results over current synthetic pathways – particularly in the facile coupling of ortho-substituted boronic acids.31
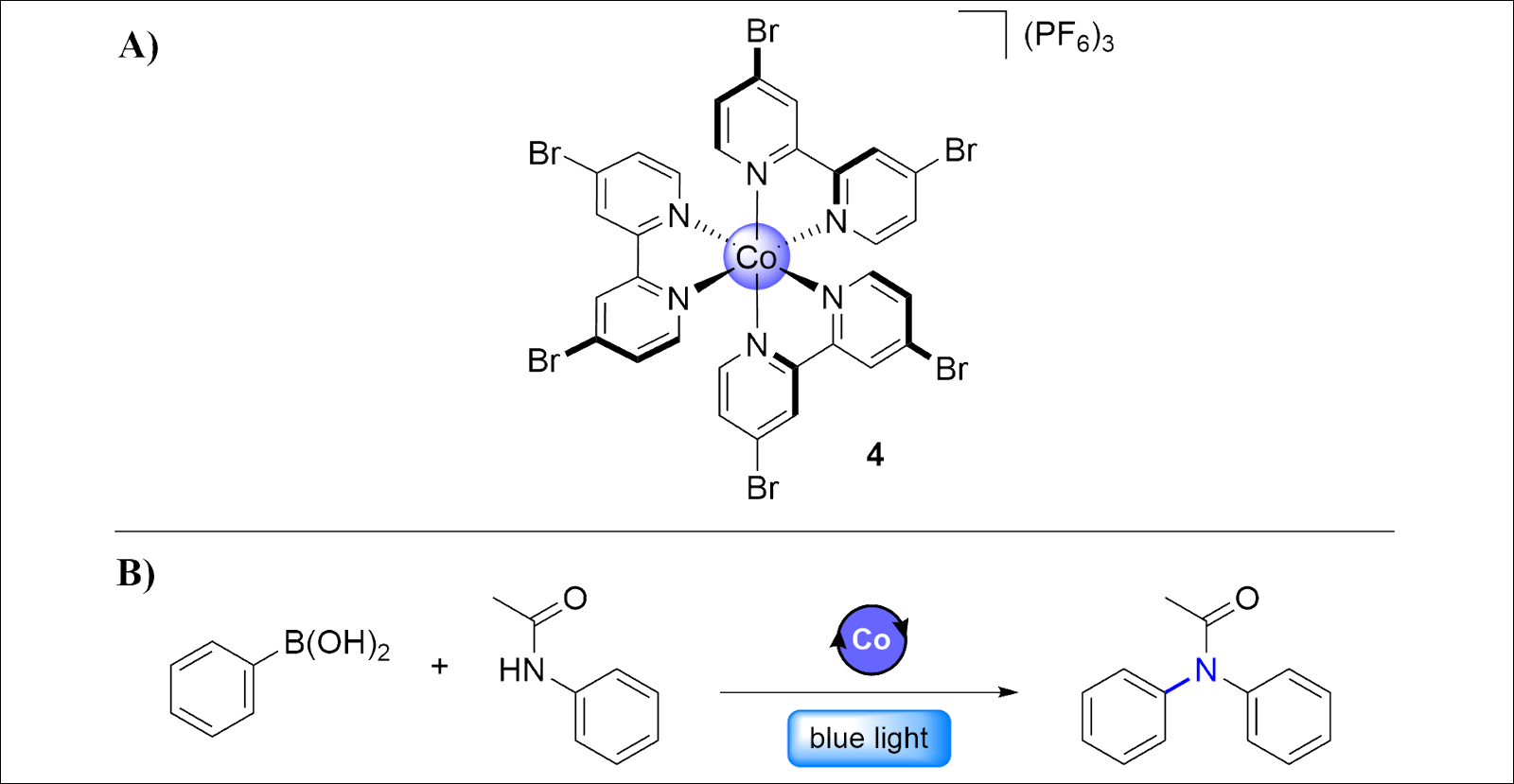
Fig. 5. (A) Structure of [Co(4,4′-Br2bpy)3]3+ (B) Representative coupling between an aryl amide and a boronic acid by 4.
Zysman-Colman and Hanan prepared CoIII complexes of each of two tridentate ligands containing diguanidylpyridine moieties (5a-b, Fig. 6a).32 These groups are strong σ-donors and contribute to the energy separation of the non-emissive ligand-field and emissive 3LMCT states.32 When compared to isoelectronic FeII complexes, complexes 5a-b feature excited state lifetimes more than 950 times longer — in the nanosecond range at room temperature.32 Redox studies demonstrated that these complexes are strong photooxidants. To test this experimentally, the mono-trifluoromethylation of four polycyclic aromatic hydrocarbons was demonstrated — the first via photosensitisation (Fig. 6c).32
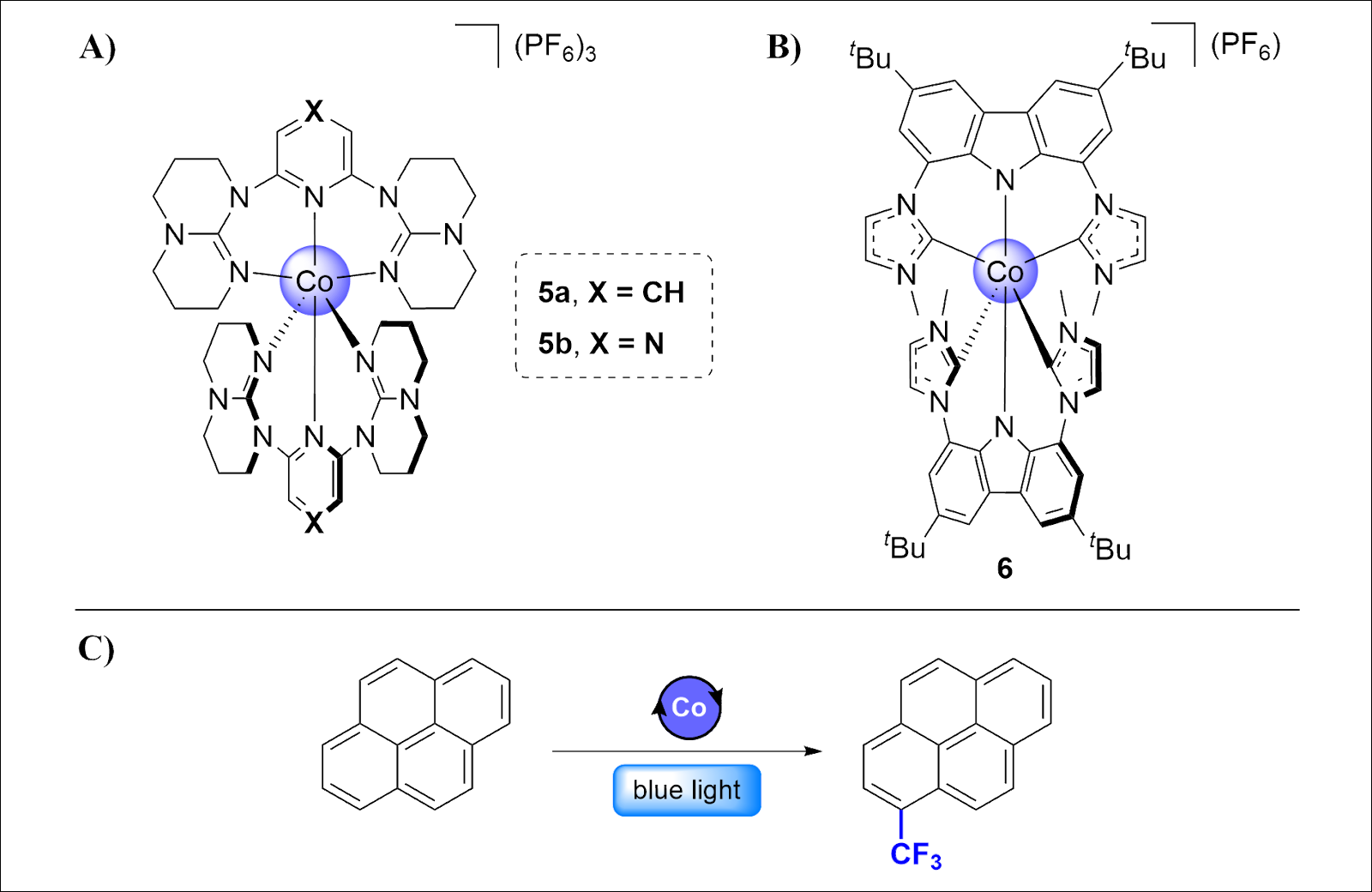
Fig. 6. (A) Zysman-Colman and Hanan’s diguanidylpyridine CoIII complex. (B) Wenger’s CoIII carbazole complex. (C) Representative trifluoromethylation of a polycyclic aromatic hydrocarbon by 5a.
More recently, the Wenger group, employing the nephelauxetic strategy developed by Herbert for FeII complexes, incorporated strongly σ-donating carbenes around an amido π-donor.22,33 The resulting complex 6 has a lifetime > 1 ns – approaching suitability for photoinduced electron transfer processes (Fig. 6b).33
Tungsten(0)
Despite being a third-row transition metal, tungsten is only about 20 times less abundant than cobalt and three orders of magnitude more abundant than ruthenium.8 In order to form stable complexes, W0 requires strongly π-accepting ligands such as carbonyls or isocyanides to stabilise its zero-valent oxidation state. Isocyanide ligands are particularly well-designed for this purpose, given that their CN donor moieties can be easily attached to a variety of substituted π-aromatic systems.
Early work on zero-valent group six metal complexes bearing monodentate isocyanides showed that such complexes of W0 exhibit 3MLCT luminescence with microsecond lifetimes, but also undergo photosubstitution reactions in the presence of nucleophiles.34 Subsequent studies showed that this process can be minimised through ligand refinement, by the installation of sterically bulky substituents that act to shield the metal centre from nucleophilic attack in the MLCT excited state. In this fashion, the Gray group reported luminescent W0 complexes bearing sterically bulky monodentate isocyanide ligands, and demonstrated their utility as powerful photoreductants (Fig. 7a).35–38 The diisopropylphenyl-substituted complex 7a (when irradiated under blue light) was shown to be capable of reducing benzophenone to its ketyl radical (Fig. 7b).36 This reaction demonstrates the potential use of W0 isocyanide complexes as powerful photoreductants, especially compared to precious metal photosensitisers such as [Ir(ppy)3] which is unable to achieve the necessary redox potentials to drive this challenging transformation. Complexes 7a-b, as well as similar complexes with more elaborated ligand designs, were later shown to be promising two-photon absorption photosensitisers, with the capability to catalyse base-promoted homolytic aromatic substitution (BHAS) reactions under near-infrared irradiation (Fig. 7c).37
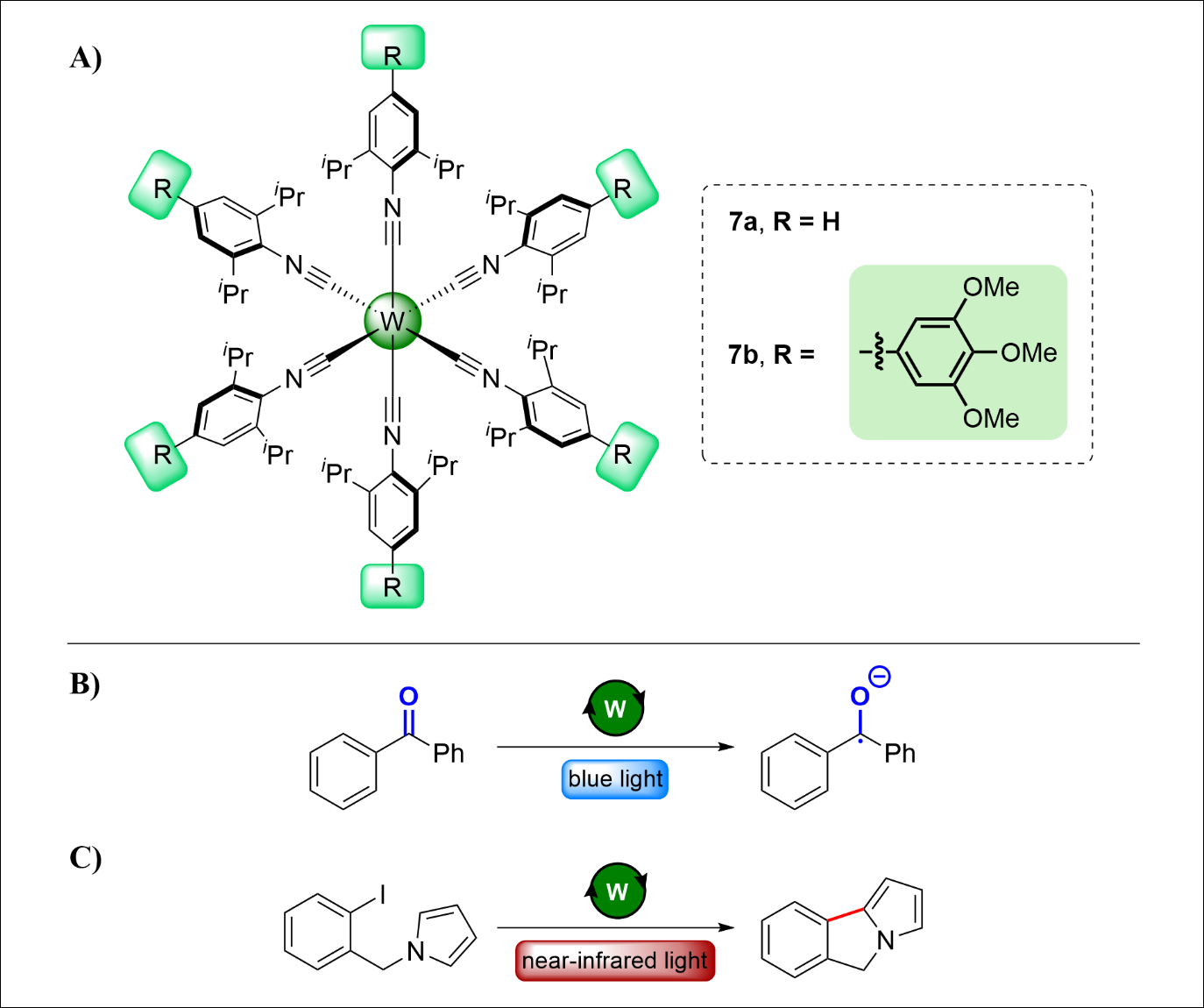
Fig. 7. (A) Structure of Gray’s W0 isocyanide complexes. (B) W0-sensitised photoreduction of benzophenone by 7a. (C) W0-catalysed BHAS reaction through two-photon absorption.
Molybdenum(0)
Moving up a row of the periodic table, molybdenum is approximately as abundant as tungsten,8 but Mo0 isocyanide complexes are inherently more photolabile. The Wenger group sought to develop (using the chelate effect) photostable Mo0 isocyanide complexes with long-lived MLCT excited states (Fig. 8a).39 The chelating diisocyanide Mo0 complex 8a indeed possesses long-lived, luminescent 3MLCT excited states that can be leveraged to perform thermodynamically challenging photoreductions, such as the rearrangement of acyl cyclopropanes to 2,3-dihydrofurans (Fig. 8b).40 It was found, however, that the terphenyl ligand scaffold, partnered with methyl substituents, leaves the metal centre partially exposed to the chemical environment, resulting in excited-state deactivation through exciplex formation. Further refinement of the ligand design was therefore desired.
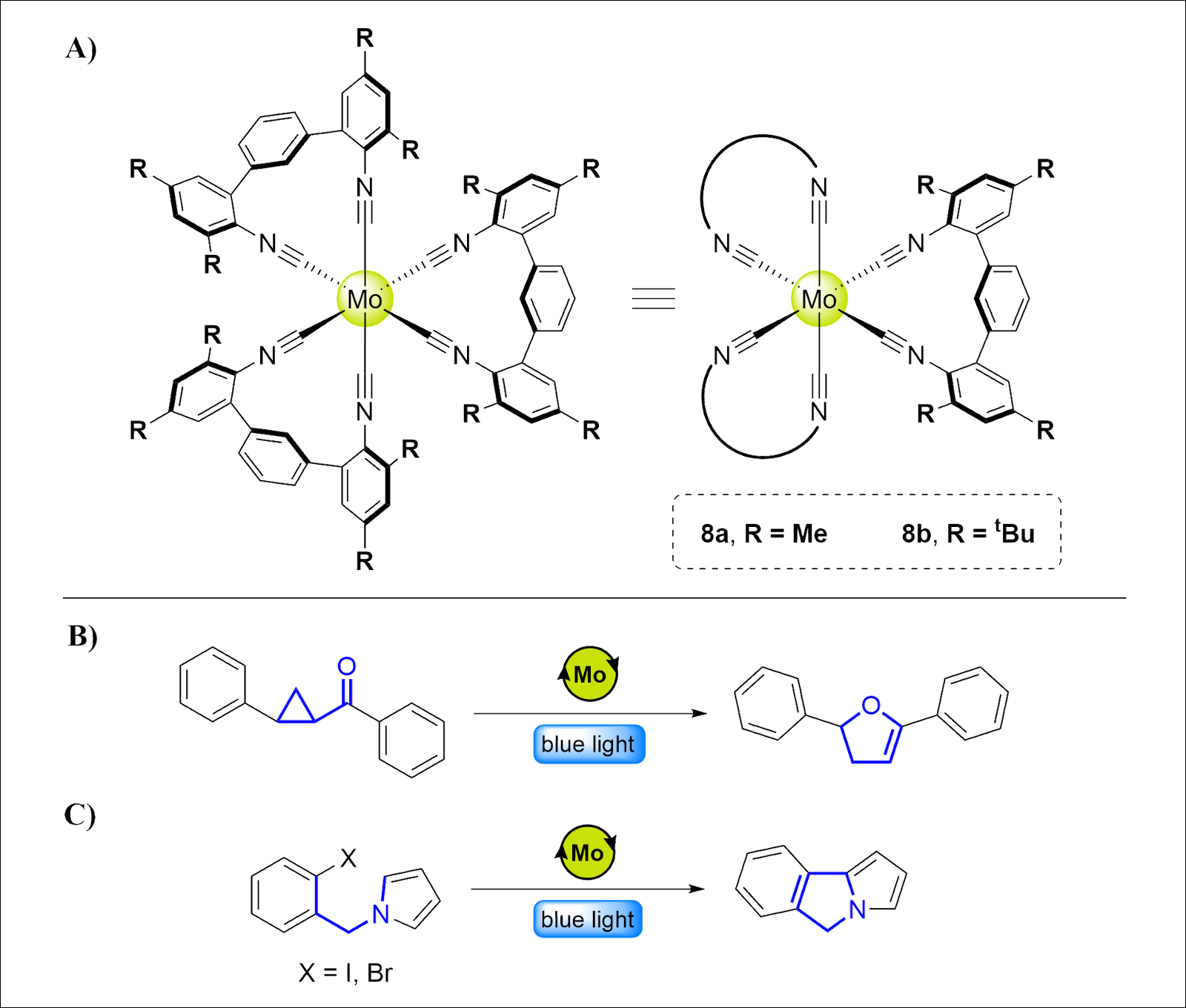
Fig. 8. (A) Wenger’s first- and second-generation Mo0 diisocyanide complexes. (B) Reductive rearrangement reaction of an acyl cyclopropane catalysed by 8a. (C) Base-promoted homolytic aromatic substitution reaction catalysed by 8b.
A second-generation complex, 8b, incorporating sterically bulkier tert-butyl groups at the relevant positions, resulted in enhanced photophysical properties and greatly enhanced efficacy as a photoredox catalyst (Fig. 8a).41 By more effectively shielding the sensitive Mo0 centre, this change had a dramatic near-tenfold effect on the 3MLCT state lifetime, with the excited-state lifetime in THF increasing to 610 ns for the second-generation complex 8b compared to 74 ns for the original 8a. The redesigned complex was shown to be an effective photoredox catalyst for BHAS reactions on a series of substrates (one example in Fig. 8c).42 We note that this same concept of shielding the metal centre from the chemical environment has also played an important role in the development of ZrIV photosensitisers.9,10
Tailored ligand design inspired by earlier chelating bidentate isocyanide ligands later resulted in a new Mo0 complex, 9, with red-shifted absorption and emission, which was leveraged for red-to-blue triplet-triplet annihilation (TTA) upconversion (Fig. 9a).43 The upconverted light was used to drive the blue-light dependent [Ru(bpy)3]2+-catalysed photoisomerisation of stilbene (Fig. 9b). Other thiophene-containing ligands, including a meridionally coordinating tridentate ligand (vide infra) were more recently studied, where it was demonstrated that thienyl-containing chelating isocyanide ligands actually impose a weaker ligand-field than those based on a terphenyl backbone, facilitating more efficient thermally-activated excited state deactivation.44
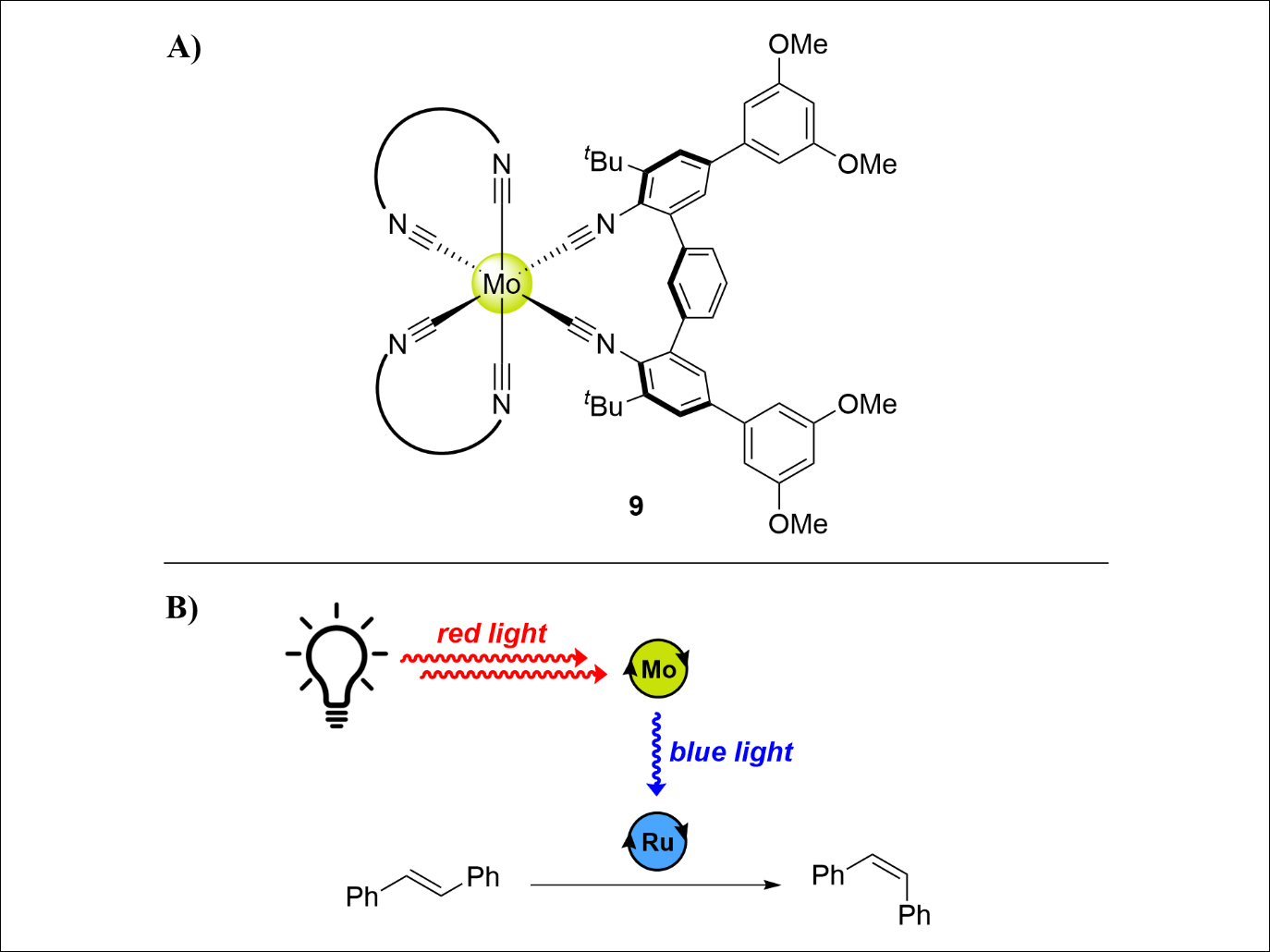
Fig. 9. (A) Wenger’s third-generation Mo0 diisocyanide complex. (B) Photoisomerisation of trans-stilbene to cis-stilbene driven by Mo0-sensitised red-to-blue TTA upconversion.
In a conceptually different approach, the Heinze group recently designed a heteroleptic tricarbonyl Mo0 complex, 10, featuring a tripodal ligand consisting of pyridine donors connected by a common quaternary carbon atom (Fig. 10a).45 Complex 10 features strong visible absorption, and red 3MLCT emission with a lifetime of up to 500 ns. 10 is two orders of magnitude more photostable than [Ru(bpy)3]2+ and, unlike the Mo0 isocyanide complexes, is air-stable. Complex 10 was shown to be an efficient photosensitiser for green-to-blue TTA upconversion, and its strong reducing power in the excited state was leveraged for the dehalogenation of chloropyrazine (Fig. 10c).
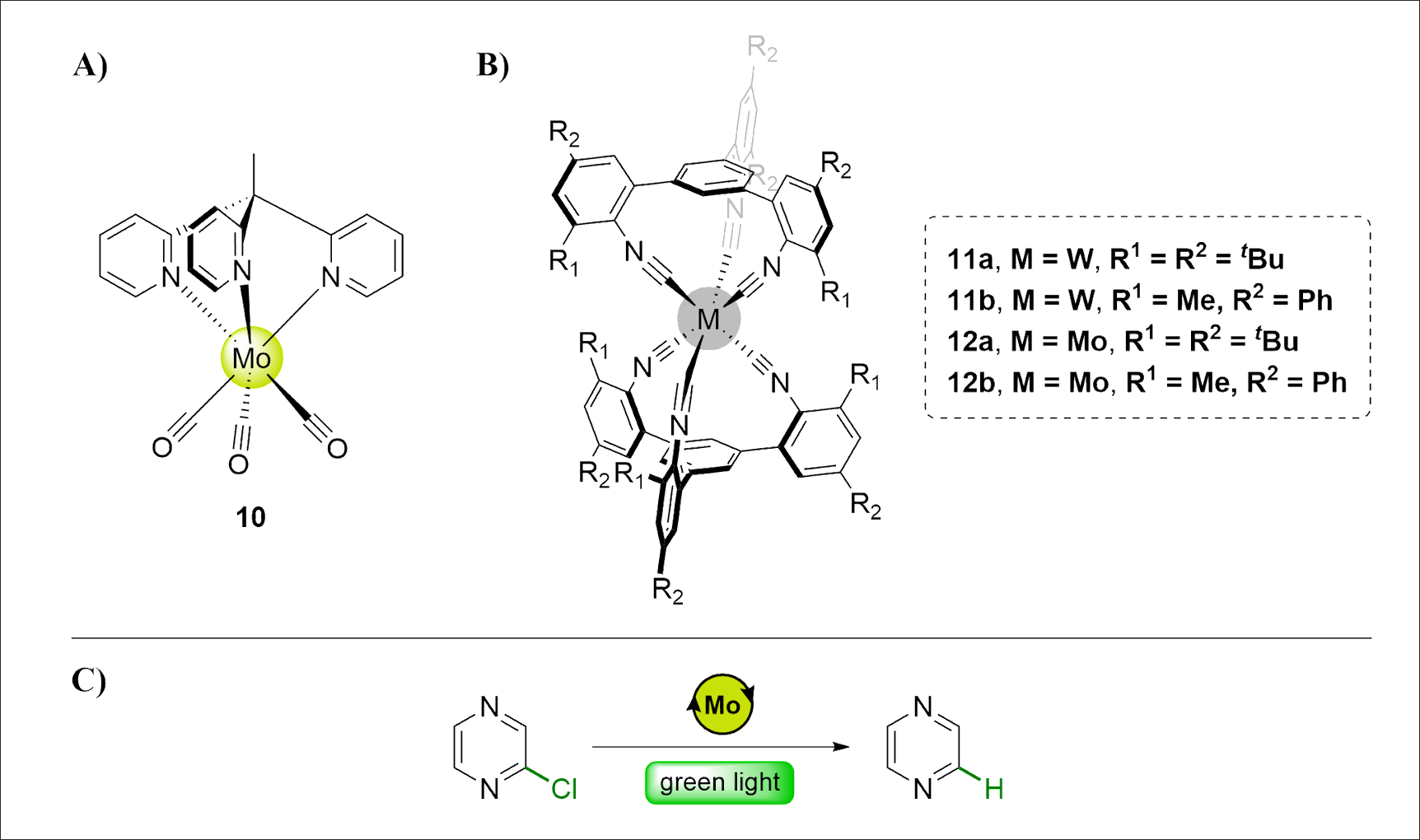
Fig. 10. Tripodal Mo0 and W0 complexes. (A) Heinze’s tricarbonyl Mo0 complex. (B) Lu’s isocyanide Mo0 and W0 complexes. (C) Dehalogenation of chloropyrazine by 10.
Combining the tripodal approach of the Heinze group with the chelating isocyanides of the Wenger group, the Lu group recently (indeed, as we were drafting this article) reported W0 (11a-b) and Mo0 (12a-b) complexes bearing facially coordinating tridentate isocyanide ligands (Fig. 10b).46 These complexes exhibit similar photophysical properties to the bidentate Mo0 complexes reported by the Wenger group, with potential application as strong photoreductants. At this stage, the photostability and catalytic performance of these innovative photosensitisers have yet to be compared to their bidentate homologues.
Chromium(0)
Given the success of the Mo0 complexes discussed above, and the high relative abundance of chromium, the Wenger group investigated Cr0 complexes bearing chelating isocyanide ligands.41,47 The unavoidable consequence of changing from Mo0 to Cr0 is again the stabilisation of ligand-field states due to the primogenic effect.14 However, the strong-field isocyanide ligands resulted in sufficient destabilisation of the ligand-field states such that the Cr0 complex 13, bearing tert-butyl substituents, exhibited 3MLCT luminescence, albeit much weaker and with a significantly shortened excited state lifetime (Fig. 11) due to partial deactivation through the ligand-field manifold.41 Despite modest photoluminescence, this is a substantial improvement compared to isoelectronic FeII complexes, representing the first example of MLCT luminescence from a first-row transition metal complex with the d6 electronic configuration, and was shown to be sufficient to sensitise TTA upconversion with anthracene.

Fig. 11. Difference in excited-state lifetimes between homologous Mo0 and Cr0 chelating isocyanide complexes.
More recently, updated ligand designs that more effectively stabilise the MLCT state have contributed to increases in excited-state Cr0 complex lifetimes (Fig. 12).48,49 These designs feature extended aromatic substituents such as mesityl (complex 14a) or pyrenyl (complexes 14b-c) groups that are better able to delocalise the negative charge on the ligand associated with an MLCT state. This design principle has resulted in first-row Cr0 isocyanide complexes with excited-state lifetimes that approach 50 ns in the case of complex 14b—a dramatic improvement on the design that incorporates a less extended π system, and a marked improvement on isoelectronic FeII complexes.
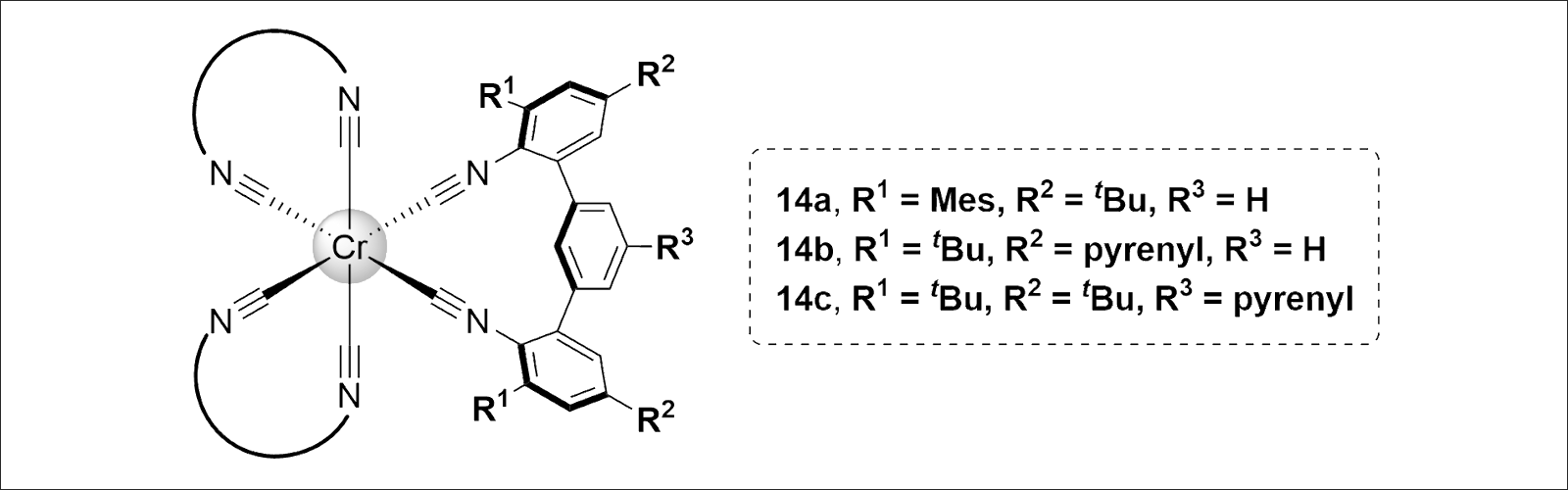
Fig. 12. Structures of Cr0 complexes bearing Wenger-type isocyanide ligands.
Manganese(I)
Despite the promise of the aforementioned Mo0 and Cr0 complexes, these compounds have a tendency to be unstable in the presence of oxygen owing to the low valencies of their metal centres. Although this can be counterbalanced somewhat by ligand design factors such as strong π-acceptor character and sterically bulky substituents to shield the metal centre,41,47 these zero-valent complexes are still usually susceptible to degradation when handled in air for longer periods of time.
Isoelectronic MnI, therefore, represents an attractive target for chelating isocyanide ligands, given that manganese is the third most abundant transition metal in the earth’s crust with MnI being much less sensitive to oxidation.8 This reduced sensitivity arises due to the higher oxidation potential of MnI, which also has the consequence of increasing the MLCT energy. Although this lessens the absorption profile of the complex in the visible spectrum, it ultimately results in additional excited state energy which can be effectively used to catalyse electron- or energy-transfer processes.
In 2021, the Wenger group reported MnI complexes (15a-b) bearing chelating isocyanide ligands, with both electron- and energy-transfer reactivity (Fig. 13).50 These complexes consist of both bidentate (15a) and meridionally coordinating tridentate (15b) scaffolds containing alternating thienyl and substituted phenyl units, and represent the first example of 3MLCT luminescence from any MnI complex. Their photophysical properties are complex, with electron-transfer reactivity occurring from the luminescent 3MLCT state, and energy-transfer reactivity arising from electronic transitions centred on the ligands themselves. Although the excited-state lifetimes are still relatively short (< 2 ns), the higher oxidation state of MnI relative to W0, Mo0, and Cr0, means that the resulting complexes are remarkably air-stable.

Fig. 13. Structures of Wenger’s MnI chelating di- and triisocyanide complexes.
Conclusions
We have herein provided a high-level overview of the current state of the emerging field of earth-abundant photosensitisers based on d6 transition metals. We have highlighted two general design approaches for accessing photoactive MLCT excited states in such complexes:
- Destabilising ligand-field excited states in medium- to high-valent metals through a) enhancement of ligand-field strength via strongly σ-donating ligands, and b) harnessing the nephelauxetic effect by increasing metal-ligand covalency.
- Stabilising low-valent metals and simultaneously destabilising ligand-field relative to MLCT excited states through the use of strongly π-accepting isocyanide or carbonyl ligands.
Only a decade ago, the concept of replacing ubiquitously employed precious metal photosensitisers in photophysical, photochemical and photobiological applications seemed unrealisable. However, a series of recent conceptual advances has accelerated progress in this important endeavour and revitalised efforts towards realistic sustainable alternatives to precious metal photosensitisers. The promise of these approaches is exemplified by the increasing rate of new discoveries—indeed, on the verge of finalising this article, Wegeberg et al. reported yet new developments in MnI and FeII isocyanide complexes.51 Furthermore, whilst we have focused specifically on accessing MLCT excited states in d6 metals for brevity’s sake, other promising approaches are rapidly developing including LMCT excited states in ZrIV complexes,9,10 and spin-flip excited states in CrIII, VII, VIII, and MnIV complexes.17,52–55 A bright future can now be envisaged for sustainable photosensitisers based on earth-abundant metals.
References
- Arias-Rotondo, D. M.; McCusker, J. K. Chemical Society Reviews 2016, 45 (21), 5803–5820.
- Prier, C. K.; Rankic, D. A.; MacMillan, D. W. C. Chemical Reviews 2013, 113 (7), 5322–5363.
- Strieth-Kalthoff, F.; James, M. J.; Teders, M.; Pitzer, L.; Glorius, F. Chemical Society Reviews 2018, 47 (19), 7190–7202.
- Hagfeldt, A.; Boschloo, G.; Sun, L.; Kloo, L.; Pettersson, H. Chemical Reviews 2010, 110 (11), 6595–6663.
- Hockin, B. M.; Li, C.; Robertson, N.; Zysman-Colman, E. Catalysis Science & Technology 2019, 9 (4), 889–915.
- Larsen, C. B.; Wenger, O. S. Chemistry – A European Journal 2018, 24 (9), 2039–2058.
- Förster, C.; Heinze, K. Chemical Society Reviews 2020, 49 (4), 1057–1070.
- Haynes, W. M. CRC Handbook of Chemistry and Physics; CRC Press, 2014.
- Zhang, Y.; Petersen, J. L.; Milsmann, C. Journal of the American Chemical Society 2016, 138 (40), 13115–13118.
- Zhang, Y.; Lee, T. S.; Favale, J. M.; Leary, D. C.; Petersen, J. L.; Scholes, G. D.; Castellano, F. N.; Milsmann, C. Nature Chemistry 2020, 12 (4), 345–352.
- Zhang, Y.; Schulz, M.; Wächtler, M.; Karnahl, M.; Dietzek, B. Coordination Chemistry Reviews 2018, 356, 127–146.
- Romero, N. A.; Nicewicz, D. A. Chemical Reviews 2016, 116 (17), 10075–10166.
- Wenger, O. S. Chemistry – A European Journal 2019, 25 (24), 6043–6052.
- McCusker, J. K. Science 2019, 363 (6426), 484–488.
- Glaser, F.; Aydogan, A.; Elias, B.; Troian-Gautier, L. Coordination Chemistry Reviews 2024, 500, 215522.
- C. Carey, M.; L. Adelman, S.; K. McCusker, J. Chemical Science 2019, 10 (1), 134–144.
- Förster, C.; Heinze, K. Chemical Physics Reviews 2022, 3 (4), 041302.
- Chábera, P.; Kjaer, K. S.; Prakash, O.; Honarfar, A.; Liu, Y.; Fredin, L. A.; Harlang, T. C. B.; Lidin, S.; Uhlig, J.; Sundström, V.; Lomoth, R.; Persson, P.; Wärnmark, K. The Journal of Physical Chemistry Letters 2018, 9 (3), 459–463.
- Kaufhold, S.; Rosemann, N. W.; Chábera, P.; Lindh, L.; Losada, I. B.; Uhlig, J.; Pascher, T.; Strand, D.; Wärnmark, K.; Yartsev, A.; Persson, P. Journal of the American Chemical Society 2021.
- Ellen Johnson, C.; Schwarz, J.; Deegbey, M.; Prakash, O.; Sharma, K.; Huang, P.; Ericsson, T.; Häggström, L.; Bendix, J.; Kumar Gupta, A.; Jakubikova, E.; Wärnmark, K.; Lomoth, R. Chemical Science 2023, 14 (37), 10129–10139.
- Braun, J. D.; Lozada, I. B.; Kolodziej, C.; Burda, C.; Newman, K. M. E.; van Lierop, J.; Davis, R. L.; Herbert, D. E. Nature Chemistry 2019, 11 (12), 1144–1150.
- Larsen, C. B.; Braun, J. D.; Lozada, I. B.; Kunnus, K.; Biasin, E.; Kolodziej, C.; Burda, C.; Cordones, A. A.; Gaffney, K. J.; Herbert, D. E. Journal of the American Chemical Society 2021, 143 (49), 20645–20656.
- Sinha, N.; Yaltseva, P.; Wenger, O. S. Angewandte Chemie 2023, 135 (30), e202303864.
- Braun, J.; Lozada, I.; Herbert, D. Inorganic Chemistry 2020, 59, 17746.
- Dixon, I. M.; Alary, F.; Boggio-Pasqua, M.; Heully, J.-L. Dalton Transactions 2015, 44 (30), 13498–13503.
- Dixon, I. M.; Khan, S.; Alary, F.; Boggio-Pasqua, M.; Heully, J.-L. Dalton Transactions 2014, 43 (42), 15898–15905.
- Leis, W.; Argüello Cordero, M. A.; Lochbrunner, S.; Schubert, H.; Berkefeld, A. Journal of the American Chemical Society 2022, 144 (3), 1169–1173.
- Yarranton, J. T.; McCusker, J. K. Journal of the American Chemical Society 2022, 144 (27), 12488–12500.
- Alowakennu, M. M.; Ghosh, A.; McCusker, J. K. Journal of the American Chemical Society 2023, 145 (38), 20786–20791.
- Banza Lubaba Nkulu, C.; Casas, L.; Haufroid, V.; De Putter, T.; Saenen, N. D.; Kayembe-Kitenge, T.; Musa Obadia, P.; Kyanika Wa Mukoma, D.; Lunda Ilunga, J.-M.; Nawrot, T. S.; Luboya Numbi, O.; Smolders, E.; Nemery, B. Nature Sustainability 2018, 1 (9), 495–504.
- Chan, A. Y.; Ghosh, A.; Yarranton, J. T.; Twilton, J.; Jin, J.; Arias-Rotondo, D. M.; Sakai, H. A.; McCusker, J. K.; MacMillan, D. W. C. Science 2023, 382 (6667), 191–197.
- Pal, A. K.; Li, C.; Hanan, G. S.; Zysman-Colman, E. Angewandte Chemie International Edition 2018, 57 (27), 8027–8031.
- Sinha, N.; Pfund, B.; Wegeberg, C.; Prescimone, A.; Wenger, O. S. Journal of the American Chemical Society 2022.
- Mann, K. R.; Gray, H. B.; Hammond, G. S. Journal of the American Chemical Society 1977, 99 (1), 306–307.
- Sattler, W.; Ener, M. E.; Blakemore, J. D.; Rachford, A. A.; LaBeaume, P. J.; Thackeray, J. W.; Cameron, J. F.; Winkler, J. R.; Gray, H. B. Journal of the American Chemical Society 2013, 135 (29), 10614–10617.
- Sattler, W.; Henling, L. M.; Winkler, J. R.; Gray, H. B. Journal of the American Chemical Society 2015, 137 (3), 1198–1205.
- Fajardo, J. Jr.; Barth, A. T.; Morales, M.; Takase, M. K.; Winkler, J. R.; Gray, H. B. Journal of the American Chemical Society 2021, 143 (46), 19389–19398.
- Barth, A. T.; Fajardo, J. Jr.; Sattler, W.; Winkler, J. R.; Gray, H. B. Accounts of Chemical Research 2023, 56 (14), 1978–1989.
- Jin, T.; Wagner, D.; Wenger, O. S. Angewandte Chemie International Edition 2023, e202314475.
- Büldt, L. A.; Guo, X.; Prescimone, A.; Wenger, O. S. Angewandte Chemie International Edition 2016, 55 (37), 11247–11250.
- Büldt, L. A.; Wenger, O. S. Angewandte Chemie International Edition 2017, 56 (21), 5676–5682.
- Herr, P.; Glaser, F.; Büldt, L. A.; Larsen, C. B.; Wenger, O. S. Journal of the American Chemical Society 2019, 141 (36), 14394–14402.
- Bilger, J. B.; Kerzig, C.; Larsen, C. B.; Wenger, O. S. Journal of the American Chemical Society 2021, 143 (3), 1651–1663.
- Herr, P.; Schwab, A.; Kupfer, S.; Wenger, O. S. ChemPhotoChem 2022, 6 (8), e202200052.
- Kitzmann, W. R.; Bertrams, M.-S.; Boden, P.; Fischer, A. C.; Klauer, R.; Sutter, J.; Naumann, R.; Förster, C.; Niedner-Schatteburg, G.; Bings, N. H.; Hunger, J.; Kerzig, C.; Heinze, K. Journal of the American Chemical Society 2023, 145 (30), 16597–16609.
- Yue, M.; He, J.; Zou, C.; Chang, X.; Lu, W. Inorganic Chemistry 2024.
- Büldt, L. A.; Guo, X.; Vogel, R.; Prescimone, A.; Wenger, O. S. Journal of the American Chemical Society 2017, 139 (2), 985–992.
- Wegeberg, C.; Häussinger, D.; Wenger, O. S. Journal of the American Chemical Society 2021, 143 (38), 15800–15811.
- Sinha, N.; Wegeberg, C.; Häussinger, D.; Prescimone, A.; Wenger, O. S. Nature Chemistry 2023, 15 (12), 1730–1736.
- Herr, P.; Kerzig, C.; Larsen, C. B.; Häussinger, D.; Wenger, O. S. Nature Chemistry 2021, 13 (10), 956–962.
- Wegeberg, C.; Häussinger, D.; Kupfer, S.; Wenger, O. S. Journal of the American Chemical Society 2024.
- Dorn, M.; Hunger, D.; Förster, C.; Naumann, R.; van Slageren, J.; Heinze, K. Chemistry – A European Journal 2023, 29 (9), e202202898.
- Dorn, M.; Kalmbach, J.; Boden, P.; Päpcke, A.; Gómez, S.; Förster, C.; Kuczelinis, F.; Carrella, L. M.; Büldt, L. A.; Bings, N. H.; Rentschler, E.; Lochbrunner, S.; González, L.; Gerhards, M.; Seitz, M.; Heinze, K. Journal of the American Chemical Society 2020, 142 (17), 7947–7955.
- Bürgin, T. H.; Glaser, F.; Wenger, O. S. Journal of the American Chemical Society 2022, 144 (31), 14181–14194.
- East, N. R.; Naumann, R.; Förster, C.; Ramanan, C.; Diezemann, G.; Heinze, K. Nature Chemistry 2024, 1–8.