Introduction
Nature provides the inspiration to design synthetic receptor mimics for molecular recognition of guests of complementary size, shape and dimensions. The development of such synthetic receptors facilitates a better understanding of the molecular mechanism of receptor site and enzyme activity through an in vitro approach. In the 1980s, Nobel laureate Donald J. Cram reached a milestone with the synthesis of a carcerand derived from covalent bond formation. Since then, more such synthetic receptors have come into existence. To mimic metalloenzymes, the development of a synthetic receptor bearing a metal centre is indispensable, where synthetic ligands can duplicate the in vivo scenario for a working metal. The molecules possess a predefined cavity that can act as an optimum building block for the synthesis of these biomimics as metal complexes and coordination cages. In this review article, aspects of cavity-based metal assembly and propagation into subspecies are described.
Metal complexes on cavity platforms
A functionalisable, well-defined molecular cavity architecture could be considered the optimum candidate for designing metal complexes. The control of metal ion nuclearity and the relative position of the metal complex on the cavity platform are generally achieved with a rationally functionalised cavity molecule. Thus, calixarene, cyclodextrin (CD), cyclotriveratrylene (CTV), and resorcin[4]arene-derived cavitands are commonly used as cavity molecules to develop potential ligands for metal complexation. In the case of water solubility, cyclodextrins stand out with high water solubility, which favours the formation of metal complexes as molecular receptors in aqueous solution. On the other hand, parent calixarenes, cyclotriveratrylenes, and resorcin[4]arene-derived cavitands are mostly soluble in organic solvents.
Calixarene-based metal complexes
Among all calixarenes, calix[4]arene has gained attention for use as a molecular platform for metal binding due to the well-explored functionalisation chemistry of calix[4]arene. Reinhoudt et al. exploited calix[4]arene-based Zn(II) complexes for ester hydrolysis.1 They studied bimetallic catalysis with two calix[4]arene-based metal complexes, where Zn(II) is coordinated by 2,6-bis(dimethylaminomethyl)pyridine positioned 1,2-vicinal 1, 1,3-distal 2, and 1,2,3- units 3 on the upper rim (Fig. 1). They concluded that the distance between two metal ions is critical for catalysis. With an extension of this study, three Zn(II) complexes at the 1, 2, and 3 positions evidenced catalysis in ester cleavage. After one year, Reinhoudt and colleagues reported calix[4]arene-derived ligands functionalised with [12]aneN3 on 1,2-vicinal 4, 1,3-distal 5, and 1,2,3-positions 6 of the upper rim, which could coordinate with zinc(II) and copper(II) (Fig. 1). The synthesised metal complexes were investigated for catalytic activity for phosphodiester cleavage.2
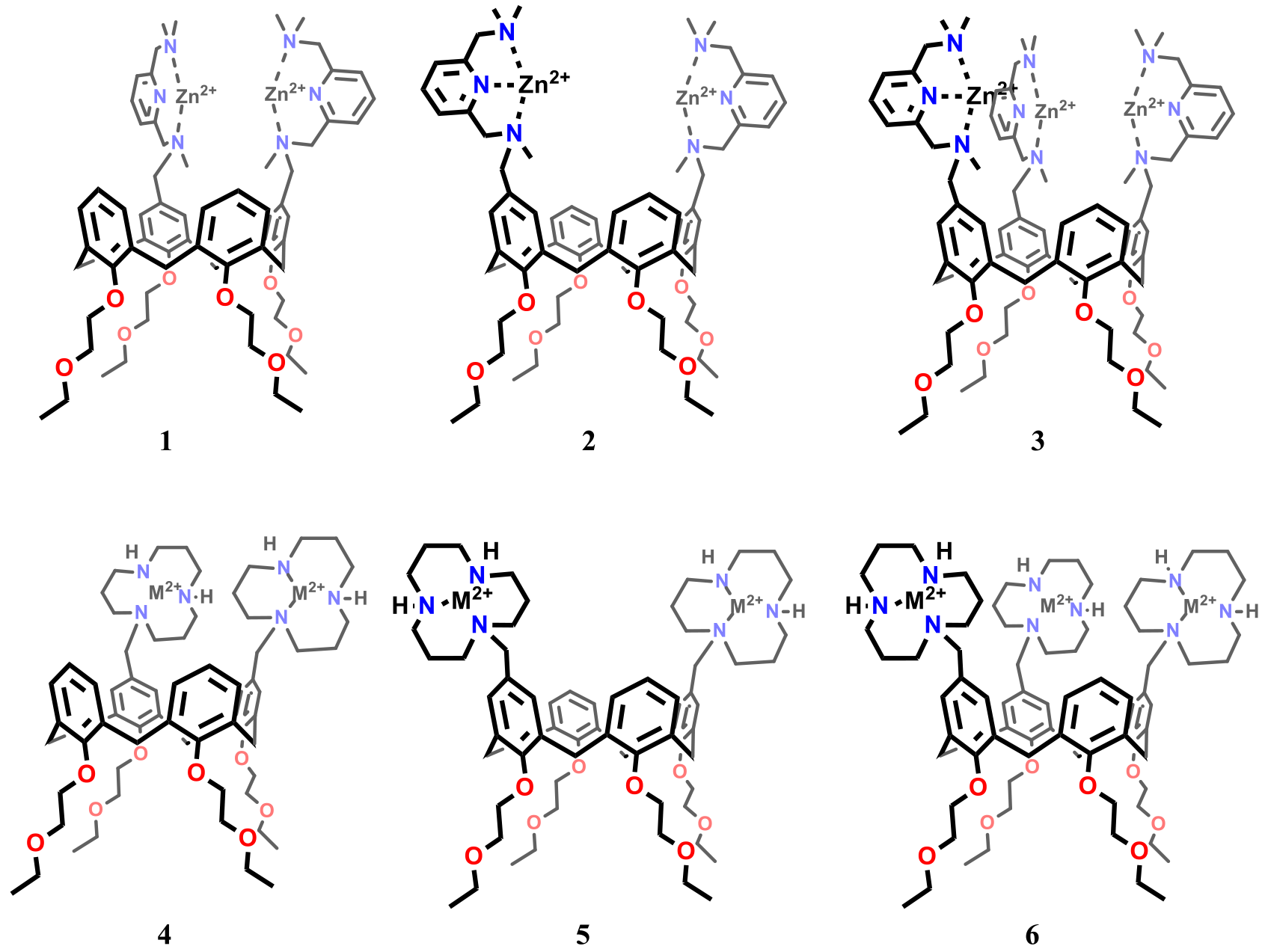
Fig. 1. The metal complexes synthesised by Reinhoudt et al. with calix[4]arene decorated with 2,6-bis(dimethylaminomethyl)pyridine or [12]aneN3.
Besides calix[4]arene, calix[6]arene can be functionalised at alternate 1-, 3-, and 5- positions of the upper rim, which facilitate the incorporation of electron donor moieties (like imidazole) to coordinate with the metal centre. The conformational flexibility of calix[6]arene 7 helps to adopt several relative conformations. In a complexation reaction with zinc(II) or copper(II), calix[6]arene adopts a funnel-like conformation. For complexation with a zinc(II) metal centre 8, calix[4]arene adopts a pseudo tetrahedral geometry consisting of an electron donating group and guest ligand inside the cavity (Fig. 2).
The guest ligand could be an exchangeable primary amine, nitrile, amide, or alcohol; the affinity not only depends on coordination with the metal centre but also on the affinity toward hydrogen bond formation and CH–π interaction inside the calix[6]arene cavity. The selectivity of guest-binding was achieved with consideration of critical factors like donor ability of the guest (e.g. an amine is a considerably better guest compared to an alcohol), steric hindrance and shape of guest (e.g. a primary amine has less steric hindrance than secondary amines and linear molecules are accommodated suitably inside the cavity).3
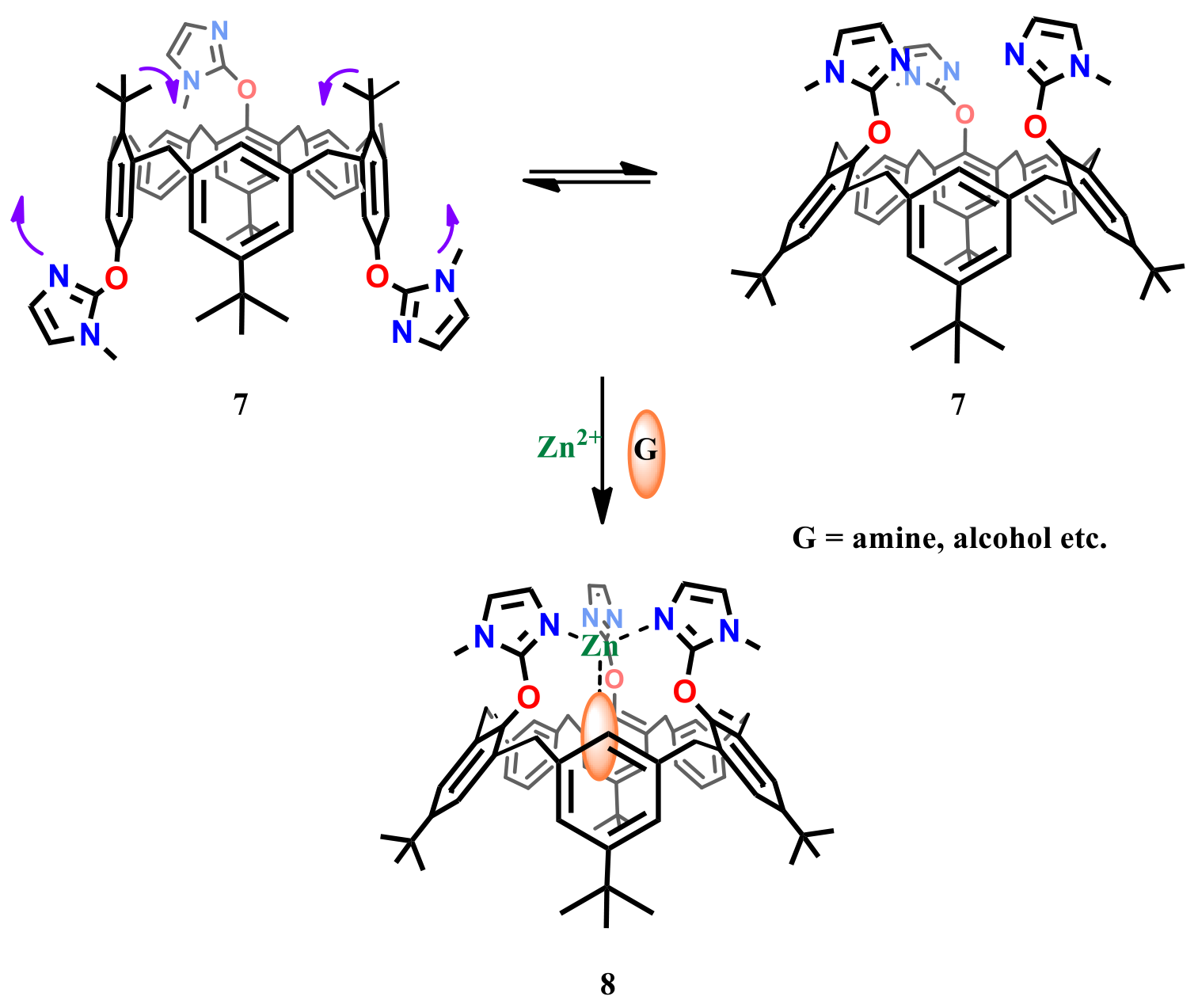
Cyclotriveratrylenes (CTV)-based metal complexes
Collet et al. reported two novel metal complexes with an n-propyl thiol and a m-substituted benzene thiol derivative of a C3-symmetric cyclotriveratrylene (CTV). The equimolar reaction between a [Fe4S4] cluster and one tridentate CTV ligand furnishes the desired metal complex in quantitative yield.4 In one study, Nolte et al. disclosed that a metal complex obtained in a reaction between [Fe4S4Cl4]2- and tri-thiol-CTV derivative, with a chloride ligand placed inside the cavity (9). On the other hand, when [Fe4S4(StBu)4]2- is used as a metal precursor, a bulky t-butyl group is coordinated from outside (10) (Fig. 3).5
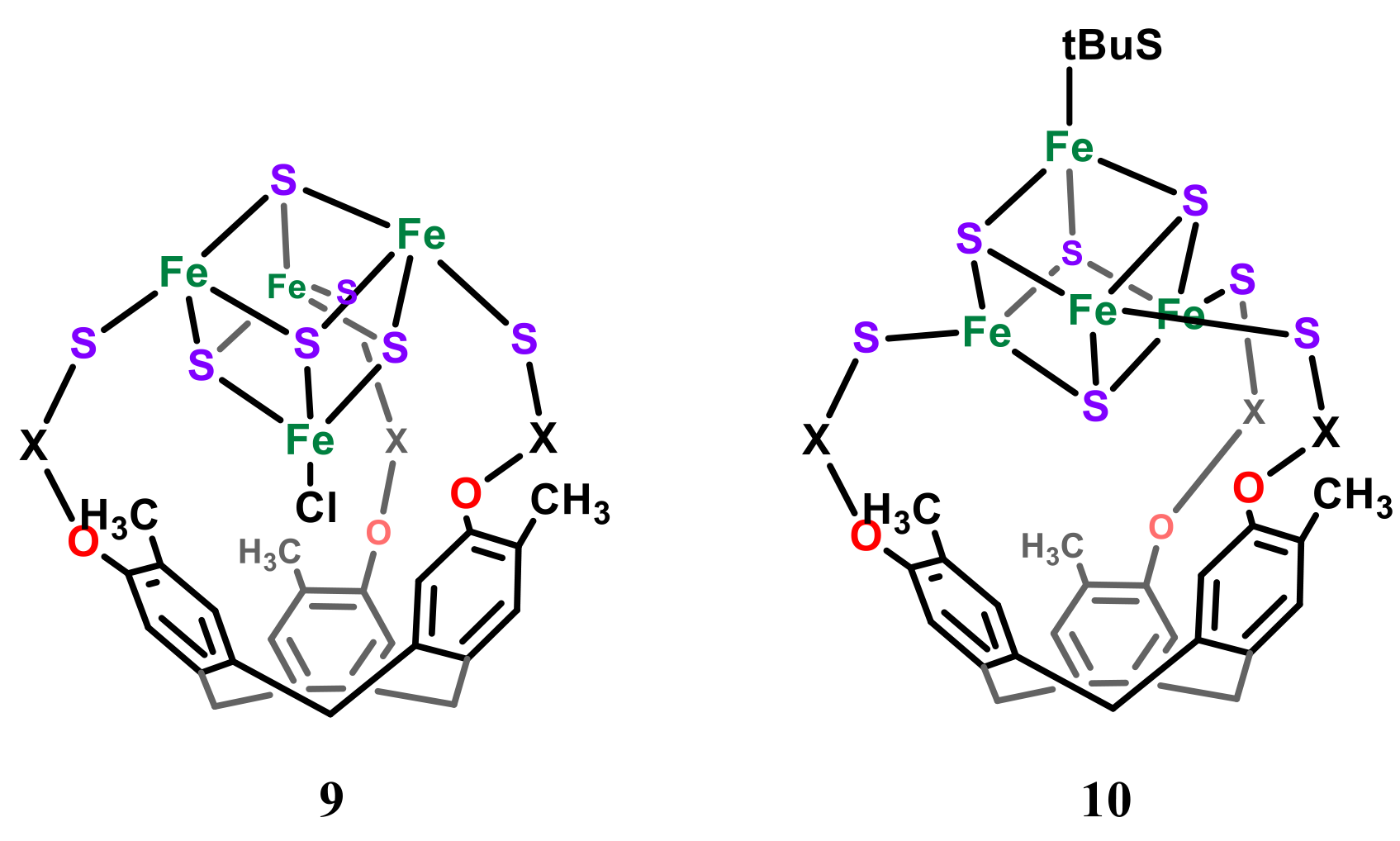
Resorcin[4]arene-derived cavitand-based metal complexes
A distinct library of cavitand-based mono- and polymetallic complexes has been prepared. To obtain a cavitand-based metal complex, the initial step is the rational modification of cavitand to act as anchor(s) for coordinating a metal centre. In some cases, the metal embedded in the cavity influences the guest recognition property of the cavitands while in other cases the metal itself changes the property by being embedded. Some early examples were reported by Puddephatt and his research group6 by developing phosphonito-cavitand and phosphinito-resorcin[4]arene multidentate ligands.
Phosphonito-cavitand and phosphinito-resorcin[4]arene assembled with Au(I) and Pt(II), respectively, to give metal complexes. The X-ray crystal structure of the phosphonito-cavitand tetragold complex clearly shows three out of four AuCl units placed on the upper rim of a phosphonito-cavitand and the fourth AuCl embedded inside the cavity (Fig. 4a). Following this line of research, Puddephatt and colleagues7 exploited phosphonito-cavitand derived tetracopper(I) (Fig. 4b) and tetrasilver(I) (Fig. 4c) complexes and their halide scavenging properties as well as reactivity towards halide replacement. In 1997, Puddephatt et al.8 described the cation recognition property of the previously reported halide inclusion metal complex. This study revealed cations like Hg and Pb (Fig. 4d) formed the dimeric metal complex. With the development of various derivatives of phosphonic-cavitand, more similar types of metal complexes were prepared by coordination with various metal centres, e.g. gold,9 silver10-11 and copper.12
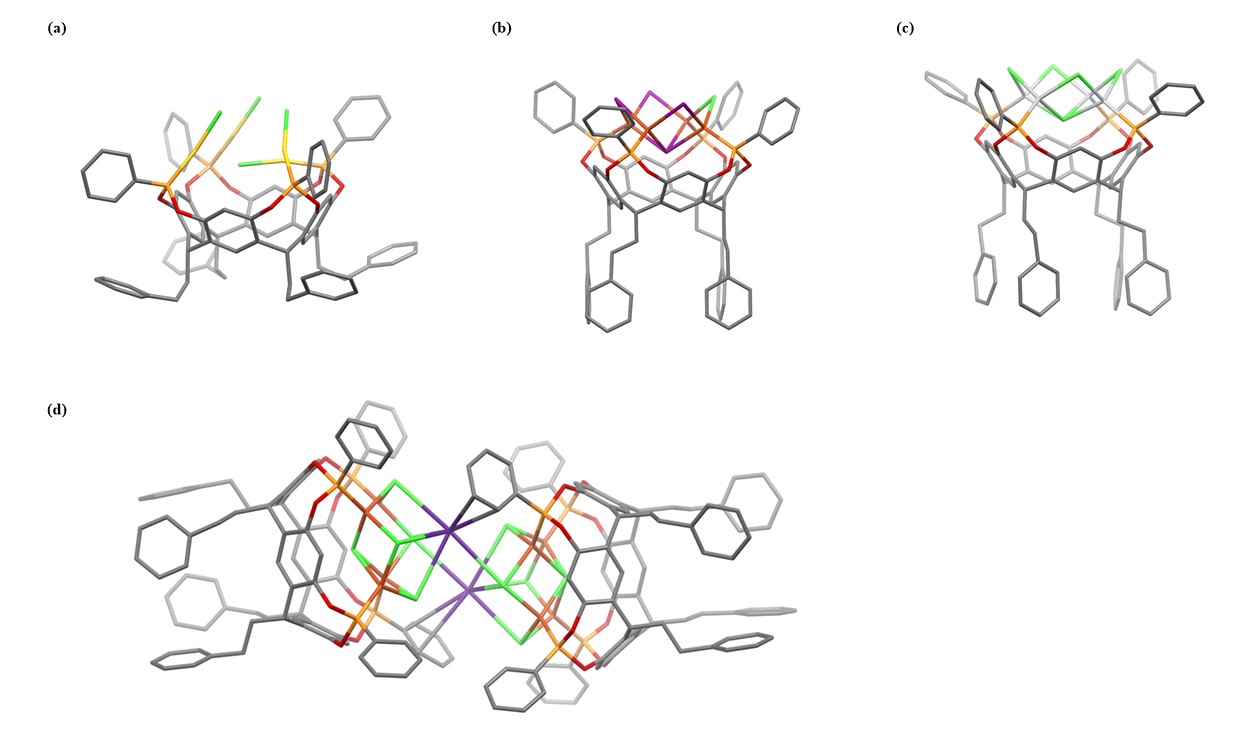
Cyclodextrin (CD)-based metal complexes
In comparison with other cavity molecules, cyclodextrin’s water solubility allows it to act as a host in aqueous media (Fig. 5a). In 2000, Kim et al.13 reported a carboxypeptidase mimic by connecting triazacyclododecane and β-cyclodextrin, following Zn(II) complexation. This metalloprotease prototype exibits hydrolysis of p-nitrophenyl acetate (PNPA) and influence the hydrolysis rate by around 300-fold. From this study, it was concluded that PNPA only bounded with CD, without coordinating with Zn(II) metal, where Zn(II) coordinated water molecule involved in hydrolysis process (Figure 5b).
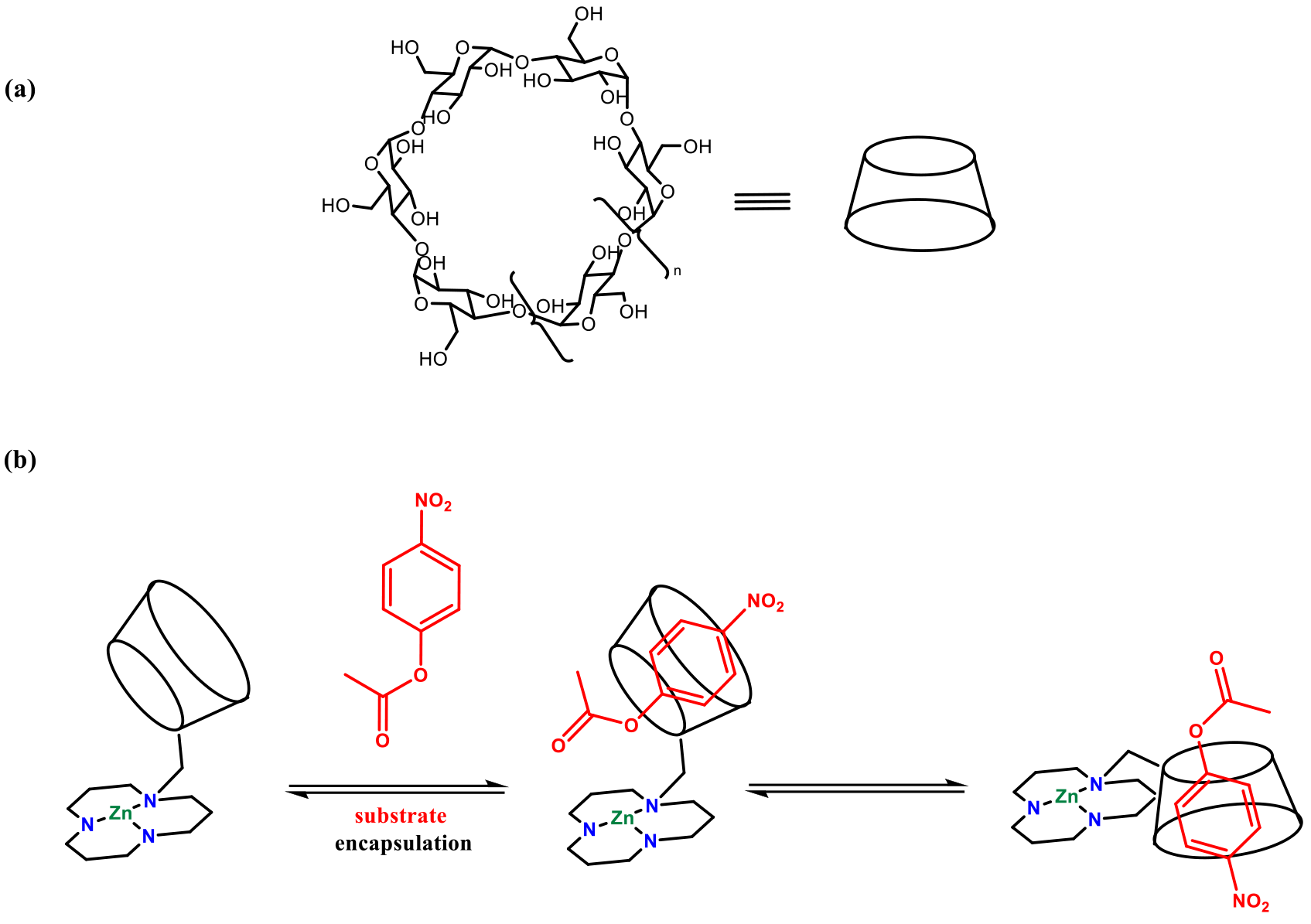
Resorcin[4]arene-derived cavitand-based coordination cages
Through their seminal work, Fujita and co-workers have opened new horizons in the application of coordination chemistry in multidisciplinary areas.14-15 In a search for size-persistent host molecules, this group reported a cis-protected Pd(II) coordination macrocycle with the 4,4’-bi-pyridyl repeating unit (Fig. 6a).14 The reported authentic framework adopted a square framework by coordinating linear ligands at a 90o coordination angle. The thermodynamically stable macrocyclic could recognise guests in an aqueous system by accommodating them between the π electron-rich eight pyridine motifs. Based upon this knowledge, Dalcanale and Jacopozzi16 successfully accomplished the first-of-its-kind co-facial cage by coordinating cavitand-based tetradentate ligands and four square planer Pd metals. The cavitand was derivatised with four cyano groups on the upper rim to anchor to Pd. Single-crystal X-ray diffraction analysis and 19F NMR revealed one out of eight trifluoromethanesulfonate counteranions are incarcerated inside the cavity, while seven stayed outside the cage.
In an extension of this research, Dalcanale et al. thoroughly investigated the factors that influence self-assembly in coordination cage synthesis (Figure 6b).17 The investigation disclosed aspects of consideration for cage synthesis: (i) the angle between the chelating ligand and the metal precursor is close to 90°, (ii) a transition metal for complexation (like Pd, Pt), (iii) preorganisation of the tetradentate cavitand and (iv) various counterions.
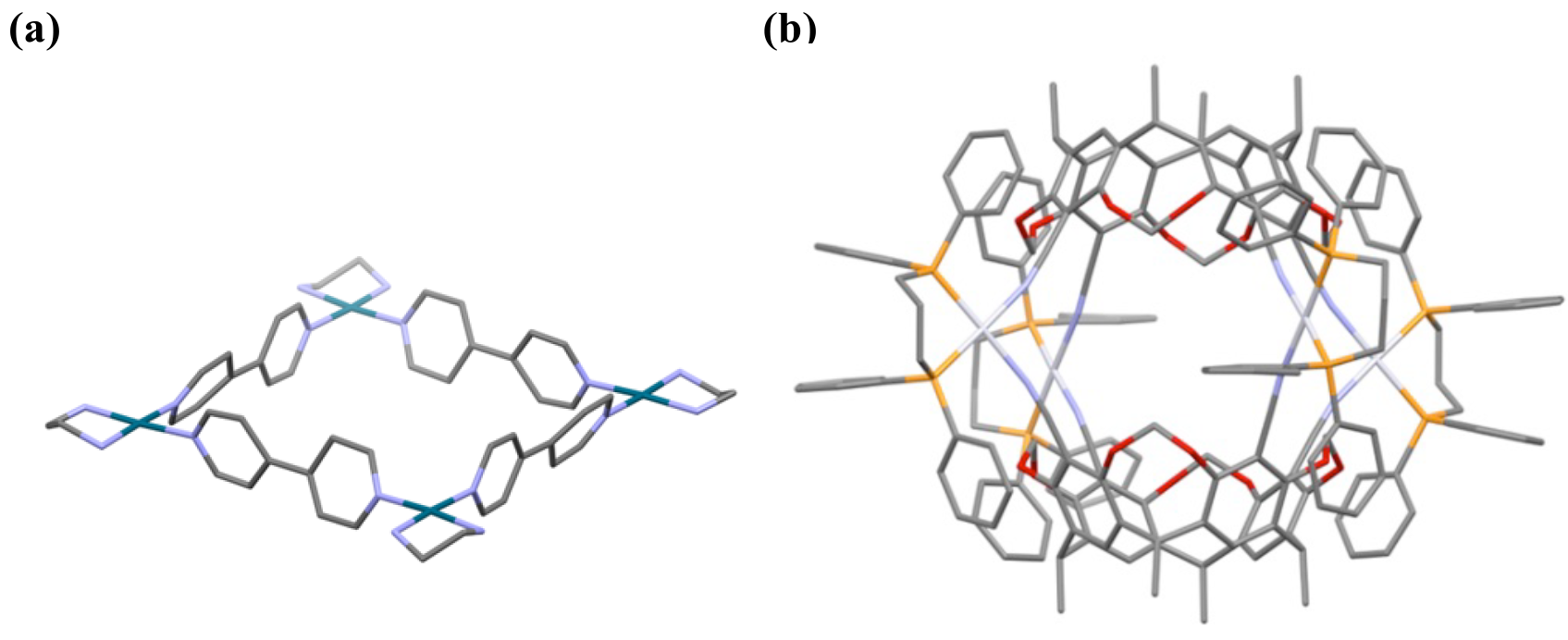
Following the Dalcanale legacy in building cavity-based coordination cages, CoCl218 and FeCl219 mediated water-soluble coordination cages (12a and 12b) were reported by Harrison et al. The decoration of the cavitand with apically-situated tetra-iminodiacetate moieties as a ligand was the first step of the reaction (Scheme 1). While FeCl2 is used as a metal source, employing a reducing agent or oxygen-free environment was essential to minimise iron oxidation. The research team disclosed that the self-assembly of the cages in aqueous media was favoured at pH > 5. In an extension of the study, encapsulation of a small guest inside the CoCl2-mediated coordination cage was investigated.20-21 The hydrophobicity as well as cavity compatibility to small organic guests act as a driving force to entrap them inside the hydrophobic cavity of the cages. It was realised that small organic guests were incarcerated during cage formation due to insolubility in aqueous media.

Extended cavity co-facial coordination cages
To accommodate larger guests inside the cavity, a cage consisting of an extended cavity is obligatory. There are two distinct approaches to obtaining an oversized coordination cage: 1) pre-coordination modification of cavitand to achieve an elongated ligand for co-facial coordination cage, or 2) synthesis by multi cavitand assembly (i.e. trimeric, tetrameric coordination cage).
The first approach was exemplified by Dalcanale and colleagues,22 when Pt metal centre derived coordination cage 14 was accomplished with extended cavitand (Scheme 2). Cage synthesis was achieved with the development of a tetrakis(4-cyanophenyl) cavitand 13 as a hemispheric ligand to coordinate. With the development of extended benzyl-connected cavitand, Dalcanale and co-workers also synthesised a cavitand decorated acetal position with a tripyridyl electron-donor group. The reaction between the tetra-pyridyl cavitand with Pd or Pt metal centre furnished to the coordination cage, endowing an ellipsoid cavity with measured volume of 840 Å3.23
Extension of the study by Dalcanale et al. revealed that the capsule has enough space to accommodate methano[60]fullerene derivatives as a 1:1 complex in CD2Cl2.24 Formation of the complex is driven by dispersion forces and π-stacking between the fullerene derivative and cavity wall. Later, a less-symmetric coordination cage was reported by Dalcanale et al. while reacting tris(4-cyanophenyl)-(4-pyridylethynyl)-cavitand, Pd(dppp)OTf2 and Pt(dppp)OTf2 in 2:3:1 ratio.25
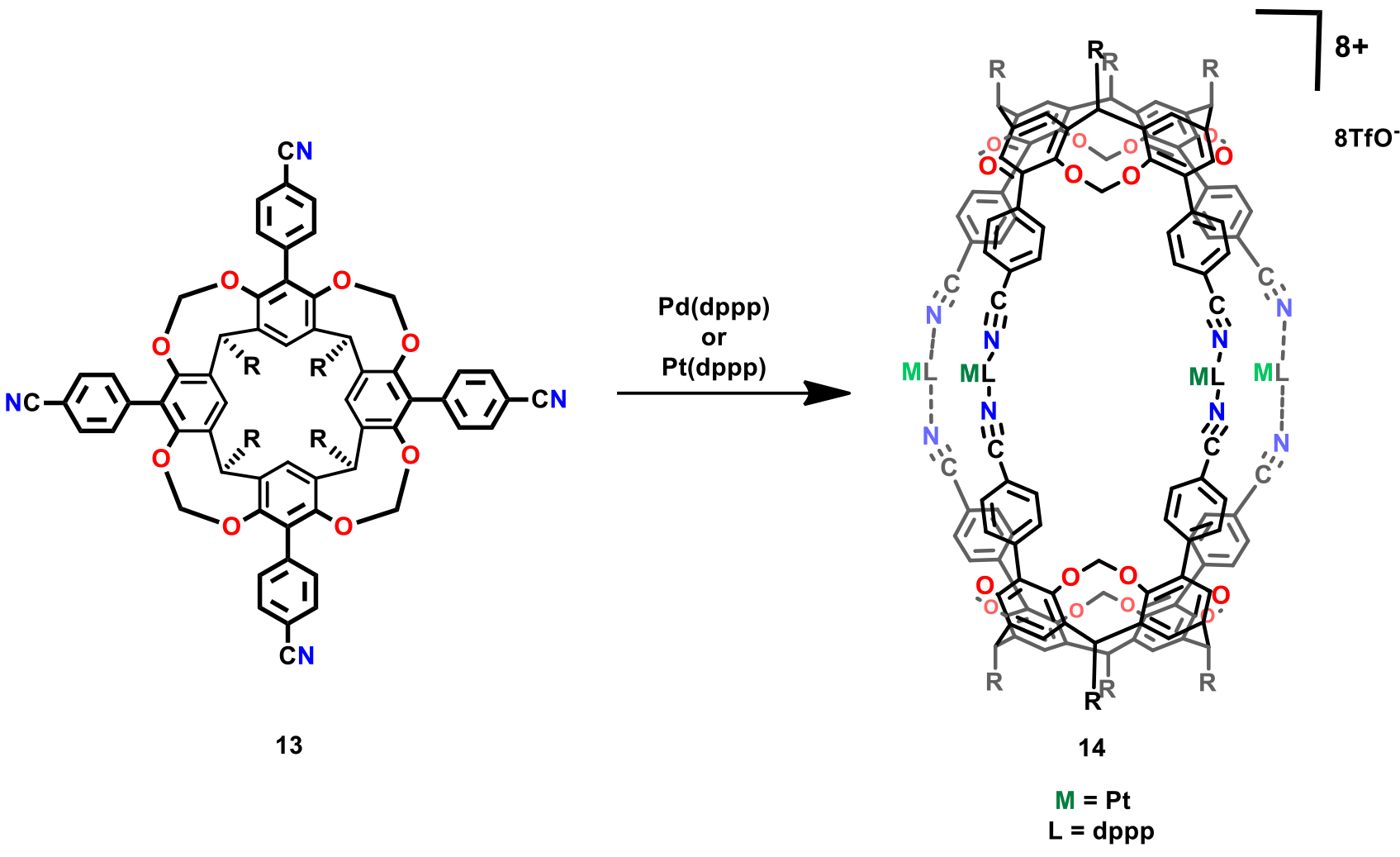
Based upon this knowledge, Kobayashi and colleagues26 demonstrated the development of homogenous and heterogenous cages with consideration of steric demand and coordination potential of the cavitand. The study found that cavitands 13 and 15 could produce homocavitand cages 17 and 18, respectively. However, equimolar self-assembly of 13 and 15 with 4 equivalent metal precursor resulted in aggregates. In contrast, cavitands 13 and 16 in equimolar co-facial coordination afforded a heterogenous coordination cage 19. The unsuccessful attempt of heterogeneous cage synthesis with 13 and 15 explained that the longer arms in 15 skewed the coordination angle to form a square planer metal complex (Fig. 7).
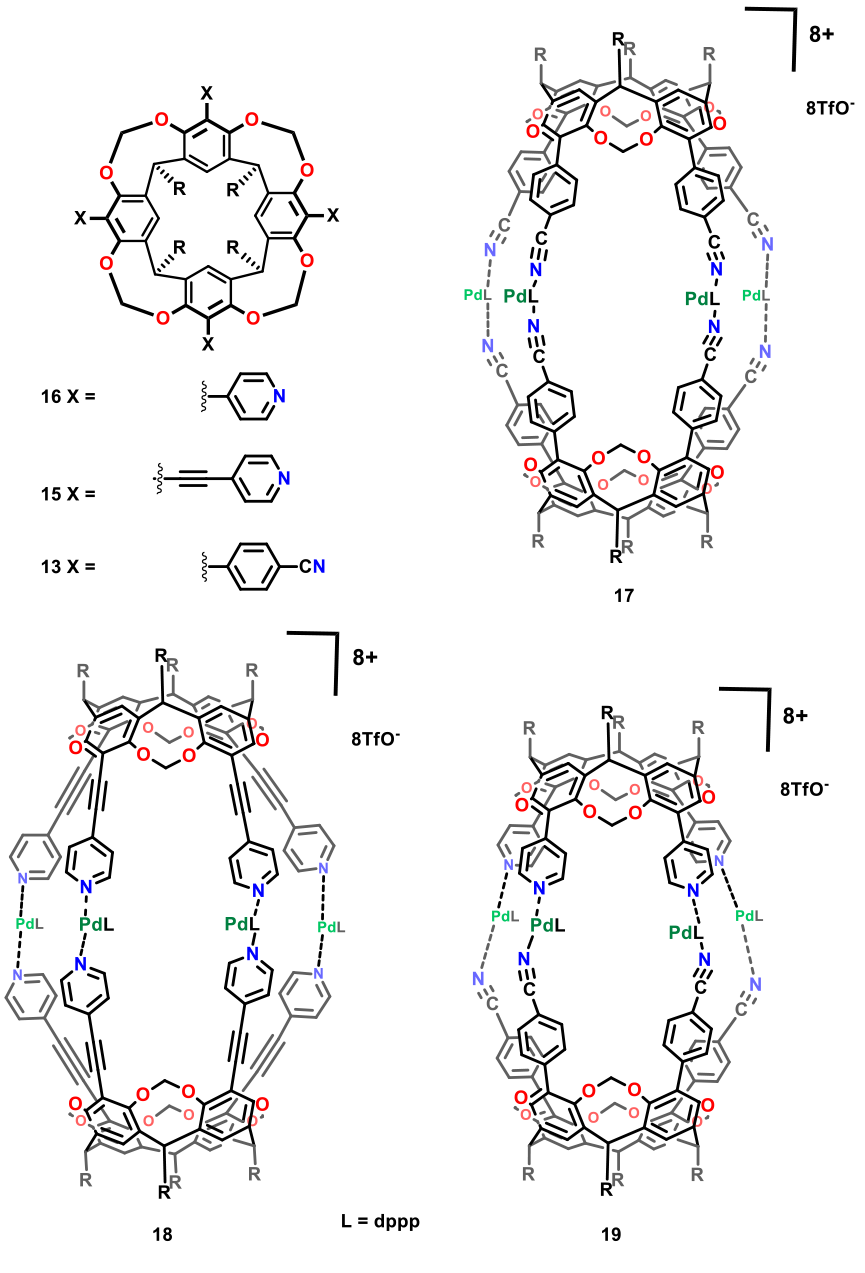
In this vein, Haino and co-workers developed more extended octadentate cavitand 20 by linking bipyridyl (bipy) moieties with phenyl spacer. Their work demonstrated the synthesis of the dimeric coordination cage 21 by coordinating two of tetra(bipyridyl) cavitand 20 with four Ag+ metal centres (Scheme 3).27 The guest inclusion ability for such an extended cavity containing cage 21 was investigated. A large aromatic compound as a guest could be incarcerated by the cage and form a thermodynamically, as well as kinetically, stable complex in CDCl3.
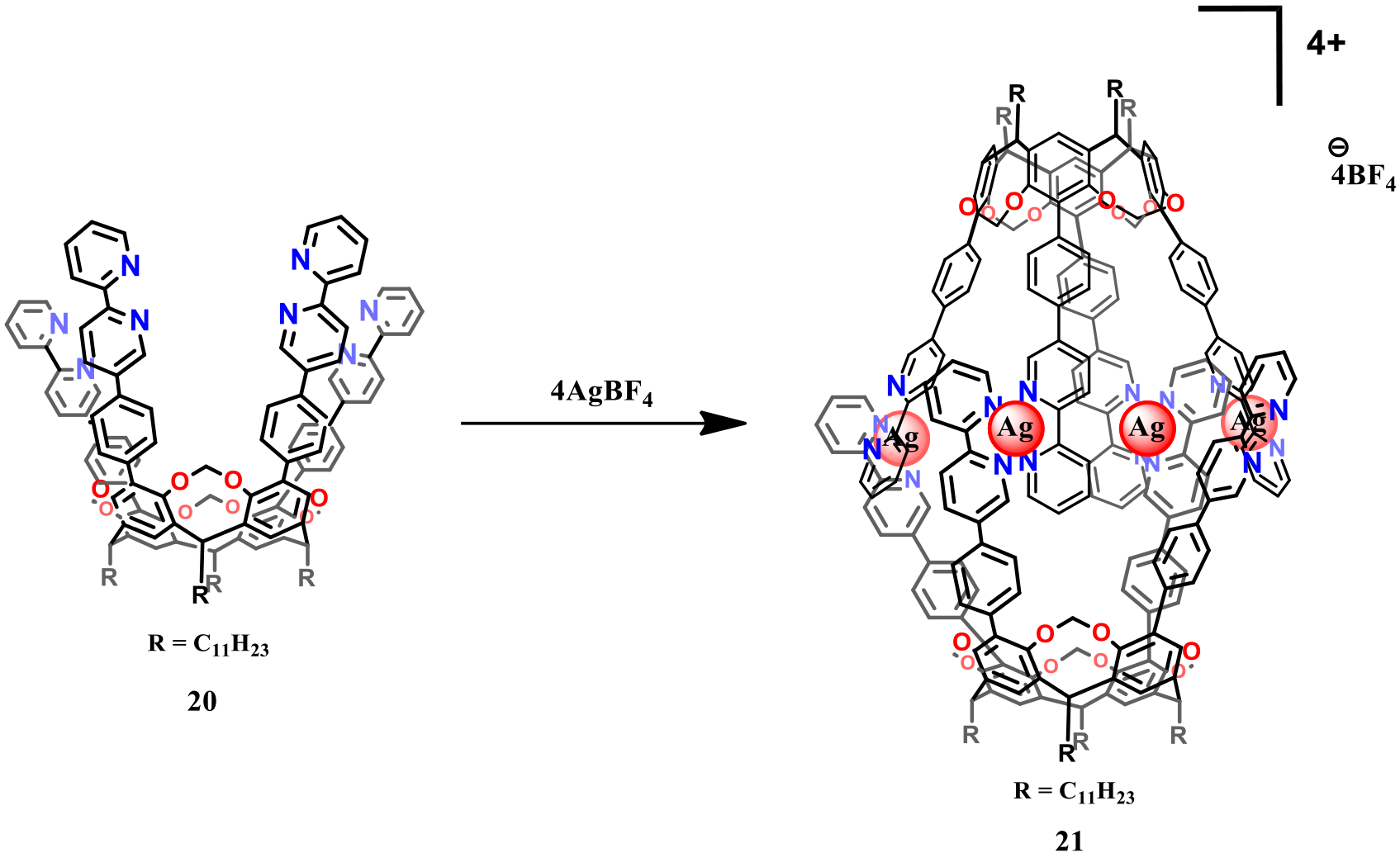
Tadokoro and colleagues28 developed a cavitand bearing four pyrimidyl groups on the upper rim. The nitrogen atoms on each pyrimidyl group directed to the cavity could complex with a metal by an inner convergent approach, and alternative nitrogen atoms on each pyrimidyl group could form metal complexes individually by an outer divergent method. The coordination with metals for such cavitands could lead to three possible outcomes: 1) a co-facial coordination cage, 2) a coordinated square array and 3) a polymeric chain.
Hong and co-worker developed a new tetradentate cavitand 22 by attaching flexible pyridine moiety to the apical position.29 Their research reported the synthesis of intramolecularly connected coordination bowls 23 with Pd or Pt metal-mediated coordination cages 23, simultaneously. Later, continuation of this study revealed the influence of the solvent system on the formation of exclusively intermolecularly coordinated cages or intramolecularly coordinated bowl molecules (Scheme 4).30
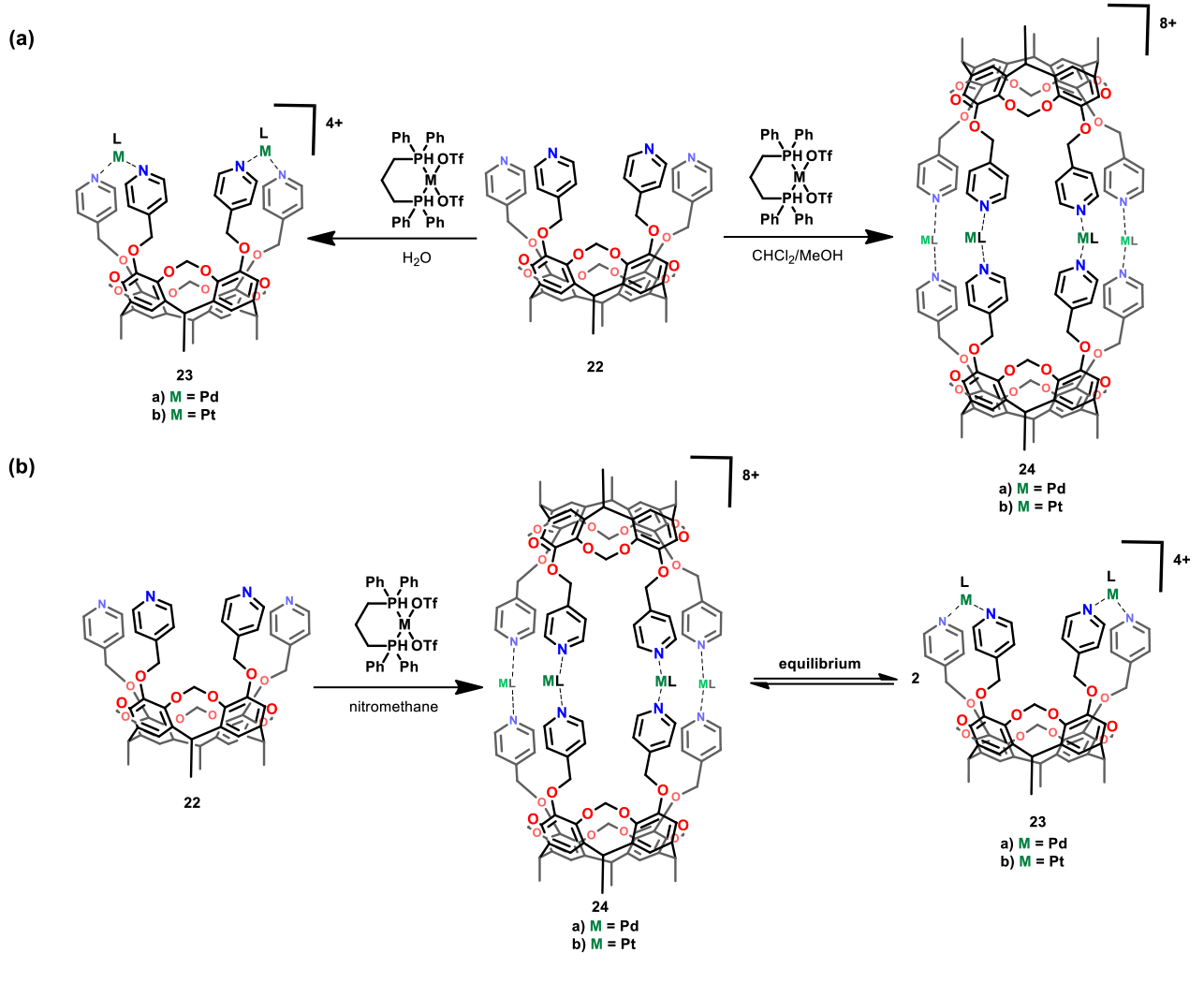
A variation of phosphonate cavitands was developed by Dalcanale et al.31 and their corresponding ditopic Re metal complex was synthesised. Both cavitands and ditopic architectures were investigated for molecular recognition and complexation properties with N-methylpyridinium salt. Their study concluded that the number of P=O groups in apical positions on cavitands was pivotal for molecular recognition and host-guest complexation.
Some hybrid coordination cages were reported with C2v-symmetrical cavitand, where cage formation was derived by coordination bond formation by connecting metal centre as well as hydrogen bond formation.32-34 In these cages, encapsulation of guests was carried out by dissociation of the weaker bond (hydrogen bond), while the stronger bond maintained the topology of the cage. Precursor cavitands of these hybrid coordination cages were designed in such a way that an electron-donating coordinating moiety and hydrogen donor-acceptor groups were placed alternatively on the apical macrocycle.
Extended cavity multi-cavitands coordination cages
In a search for larger cavity coordination cages, Beer and co-workers35 pioneeringly disclosed the synthesis of trimeric and tetrameric coordination cages. Where cavitands were ligated through coordination bonds with various d-block metal centres, their investigation revealed the metal influence in conformational arrangement of dithiocarbamate cavitands to cage synthesis. Metals like Cu(II) and Au(I) directed coordination assembly to exclusively give tetrameric cages 27. On the other hand, trimeric architecture 26 was solely obtained when Cd(II) and Zn(II) were used as metal centres (Scheme 5). Later study revealed that Ni(II), Pd(II), and Pt(II) also favoured tetrameric coordination cage synthesis.36 A guest inclusion study showed Cd(II) and Zn(II) mediated cages could accommodate fullerenes C60 and C70 in their complementary cavity and formed a 1:1 inclusion complex.37 Cu(II) based coordination cages also showed encapsulation properties with C60 and C70.
A hexameric coordination cage was reported by Holman and co-workers,38 where assembly consists of six cavitands decorated with four carboxylic acids on the upper rim and sixteen Zn(II) ions. Atwood and co-workers reported nearly the same hexameric cage.39 In contrast to other hexameric cages, pyrogallol[4]arenes was used in place of resorcin[4]arene derived cavitand and Cu(II) was employed in place of Zn(II) as the metal precursor.

A hexameric coordination cage was reported by Holman and co-workers,38 where assembly consists of six cavitands decorated with four carboxylic acids on the upper rim and sixteen Zn(II) ions. Atwood and co-workers reported nearly the same hexameric cage.39 In contrast to other hexameric cages, pyrogallol[4]arenes was used in place of resorcin[4]arene derived cavitand and Cu(II) was employed in place of Zn(II) as the metal precursor.
Conclusions
To date, a plethora of discrete cavity-based metal assemblies has been reported. With the booming host-guest chemistry, cavity-based coordination cages have transformed into more complex multi-component coordination cages from the co-facial cage. Meanwhile, cavity-based metal complexes have become predominant in biomimetics. Even after considerable exploration, the future of this field is bright and prosperous. The latent potential of cavity molecules as precursors to metal assemblies has now been understood. This is only the beginning of investigation into the rational synthesis of cavity-based metal complexes and coordination cages with different properties.
References
1. Cacciapaglia, R.; Casnati, A.; Mandolini, L.; Reinhoudt, D. N.; Salvio, R.; Sartori, A.; Ungaro, R. J. Org. Chem. 2005, 70, 5398-5402.
2. Cacciapaglia, R.; Casnati, A.; Mandolini, L.; Reinhoudt, D. N.; Salvio, R.; Sartori, A.; Ungaro, R. J. Am. Chem. Soc. 2006, 128, 12322-12330.
3. Coquière, D.; Le Gac, S.; Darbost, U.; Sénèque, O.; Jabin, I.; Reinaud, O. Org. Biomol. Chem. 2009, 7, 2485-2500.
4. Bougault, C.; Bardet, M.; Laugier, J.; Jordanov, J.; Dutasta, J.-P.; Collet, A. Supramol. Chem. 1994, 4, 139-146.
5. van Strijdonck, G. P.; van Haare, J. A.; van der Linden, J. G.; Steggerda, J.; Nolte, R. J. Inorg. Chem. 1994, 33, 999-1000.
6. Xu, W.; Rourke, J. P.; Vittal, J. J.; Puddephatt, R. J. Inorg. Chem. 1995, 34, 323-329.
7. Xu, W.; Vittal, J. J.; Puddephatt, R. J. J. Am. Chem. Soc. 1995, 117, 8362-8371.
8. Xu, W.; Vittal, J. J.; Puddephatt, R. J. Inorg. Chem. 1997, 36, 86-94.
9. Sakhaii, P.; Neda, I.; Freytag, M.; Thönnessen, H.; Jones, P. G.; Schmutzler, R. Z. Anorg. Allg. Chem. 2000, 626, 1246-1254.
10. Maslennikova, V. I.; Serkova, O. S.; Vasyanina, L. K.; Lyssenko, K. A.; Antipin, M. Y.; Nifantyev, E. E. J. Organomet. Chem. 2003, 677, 21-27.
11. Miao, S.; Yao, W.-R.; Guo, D.-S.; Zhang, Q.-F. J. Mol. Struct. 2003, 660, 159-165.
12. Zhang, Q.-F.; Adams, R. D.; Fenske, D. J. Mol. Struct. 2005, 741, 129-134.
13. Kim, D. H.; Lee, S. S. Biorg. Med. Chem. 2000, 8, 647-652.
14. Fujita, M.; Yazaki, J.; Ogura, K. J. Am. Chem. Soc. 1990, 112, 5645-5647.
15. Fujita, M. Chem. Soc. Rev. 1998, 27, 417-425.
16. Jacopozzi, P.; Dalcanale, E. Angew. Chem. Int. Ed. 1997, 36, 613-615.
17. Fochi, F.; Jacopozzi, P.; Wegelius, E.; Rissanen, K.; Cozzini, P.; Marastoni, E.; Fisicaro, E.; Manini, P.; Fokkens, R.; Dalcanale, E. J. Am. Chem. Soc. 2001, 123, 7539-7552.
18. Fox, O. D.; Dalley, N. K.; Harrison, R. G. J. Am. Chem. Soc. 1998, 120, 7111-7112.
19. Fox, O. D.; Dalley, N. K.; Harrison, R. G. Inorg. Chem. 1999, 38, 5860-5863.
20. Fox, O. D.; Leung, J. F.-Y.; Hunter, J. M.; Dalley, N. K.; Harrison, R. G. Inorg. Chem. 2000, 39, 783-790.
21. Harrison, R. G.; Burrows, J. L.; Hansen, L. D. Chem. Eur. J. 2005, 11, 5881-5888.
22. Cuminetti, N.; Ebbing, M. H.; Prados, P.; de Mendoza, J.; Dalcanale, E. Tetrahedron Lett. 2001, 42, 527-530.
23. Pirondini, L.; Bertolini, F.; Cantadori, B.; Ugozzoli, F.; Massera, C.; Dalcanale, E. Proc. Natl. Acad. Sci. U.S.A. 2002, 99, 4911-4915.
24. Pirondini, L.; Bonifazi, D.; Cantadori, B.; Braiuca, P.; Campagnolo, M.; De Zorzi, R.; Geremia, S.; Diederich, F.; Dalcanale, E. Tetrahedron 2006, 62, 2008-2015.
25. Gruppi, F.; Boccini, F.; Elviri, L.; Dalcanale, E. Tetrahedron 2009, 65, 7289-7295.
26. Kobayashi, K.; Yamada, Y.; Yamanaka, M.; Sei, Y.; Yamaguchi, K. J. Am. Chem. Soc. 2004, 126, 13896-13897.
27. Haino, T.; Kobayashi, M.; Chikaraishi, M.; Fukazawa, Y. Chem. Commun. 2005, 2321-2323.
28. Tadokoro, M.; Mizugaki, S.; Kozaki, M.; Okada, K. Chem. Commun. 2005, 1140-1142.
29. Park, S. J.; Hong, J.-I. Chem. Commun. 2001, 1554-1555.
30. Park, S. J.; Shin, D. M.; Sakamoto, S.; Yamaguchi, K.; Chung, Y. K.; Lah, M. S.; Hong, J. I. Chem. Eur. J. 2005, 11, 235-241.
31. Busi, M.; Cantadori, B.; Boccini, F.; De Zorzi, R.; Geremia, S.; Dalcanale, E. Eur. J. Org. Chem 2011, 2011, 2629-2642.
32. Yamanaka, M.; Toyoda, N.; Kobayashi, K. J. Am. Chem. Soc. 2009, 131, 9880-9881.
33. Nito, Y.; Adachi, H.; Toyoda, N.; Takaya, H.; Kobayashi, K.; Yamanaka, M. Chem. Asian J. 2014, 9, 1076-1082.
34. Yamanaka, M.; Kawaharada, M.; Nito, Y.; Takaya, H.; Kobayashi, K. J. Am. Chem. Soc. 2011, 133, 16650-16656.
35. Fox, O. D.; Drew, M. G.; Beer, P. D. Angew. Chem. Int. Ed. 2000, 39, 135-140.
36. Fox, O. D.; Cookson, J.; Wilkinson, E. J.; Drew, M. G.; MacLean, E. J.; Teat, S. J.; Beer, P. D. J. Am. Chem. Soc. 2006, 128, 6990-7002.
37. Fox, O. D.; Drew, M. G.; Wilkinson, E. J.; Beer, P. D. Chem. Commun. 2000, 391-392.
38. Ugono, O.; Moran, J. P.; Holman, K. T. Chem. Commun. 2008, 1404-1406.
39. McKinlay, R. M.; Cave, G. W.; Atwood, J. L. Proc. Natl. Acad. Sci. U.S.A. 2005, 102, 5944-5948.