Graphical Abstract1

Abstract
Transition state (TS) analogues are stable compounds resembling the chemical TS of an enzyme-catalysed reaction. TS analogues are often tightly bound by their target enzyme and thus make excellent enzyme inhibitors. The Ferrier Research Institute at Victoria University of Wellington and Professor Vern Schramm’s group at Albert Einstein College of Medicine have embarked on a three-decade-long collaboration (to date), which has aimed to fine-tune the process of TS analogue design. Over this period, the refined approach has been applied to various biologically relevant targets, resulting in the generation of potent transition state inhibitors. One particularly successful example is the creation of Mundesine®, which has gained approval in Japan for the treatment of peripheral T-cell lymphoma. This article aims to provide a high-level introduction to the process developed by the Ferrier Research Institute and Professor Vern Schramm and provide selected examples that highlight the relevance of this process to drug development.
Enzyme inhibitors
Enzymes are indispensable in biological systems, functioning to lower the activation energy of chemical transformations and increase the overall rate of reaction.2 Enzyme catalysis is featured in the vast majority of metabolic pathways, with the most efficient enzymes enhancing the rate of conversion by millions of times.2-3 It is, thus, unsurprising that enzyme activity, aberrant or otherwise, can be critical to pathological processes.2 Accordingly, enzymes are often selected as targets for drug development and enzyme inhibitors are well-represented in our current pharmaceutical toolbox.
Over the last 30 years, the Ferrier Research Institute of Victoria University of Wellington (previously of Callaghan Innovation and Industrial Research Ltd) have forged a fruitful collaboration with Prof. Vern Schramm, an enzymologist at the Albert Einstein College of Medicine in New York. Together, the two institutes have fine-tuned the process of preparing potent enzyme inhibitors by synthesising compounds that mimic the transition state (TS) of a given enzyme (see selected reviews4-7). In this way, tight-binding inhibitors have been prepared that have applications against various diseases,6 including cancer,8 gout7 and infectious diseases.4 It should be noted that it is not the intent of the authors to provide an exhaustive review of all elements of enzyme inhibitor design but instead to give a high-level overview introducing the reader to the general approach taken by the Ferrier Research Institute and Prof. Vern Schramm’s group. Accordingly, this article aims to introduce TS analogue design, providing select examples that exemplify the power of this technology.
The journey from TS blueprint to TS analogues
As early as the mid-20th century, it was proposed that enzyme rate enhancement is imparted by tight binding of the chemical TS,9 which is the point of highest energy relative to the reaction substrate(s).2 Transition state theory suggests that chemically stable mimics of the enzymatic transition state will bind to the active site tighter than the substrate by a factor that reflects the rate of catalytic enhancement (Kenz/Knonenz), usually falling between 1010 and 1015.10 Accordingly, TS analogues are often tightly bound by their target enzyme, with dissociation constants falling in the nanomolar to picomolar range (10-9 - 10-12 M).6 As the TS of an enzyme-catalysed reaction exists for just a fraction of the total reaction coordinate (femtosecond timescale),11 understanding the nature of the TS can represent a particularly challenging undertaking. Professor Vern Schramm’s team, in conjunction with the Ferrier Research Institute, have pioneered the determination and understanding of enzymatic TSs, using kinetic isotope effects (KIEs) to extract information on the changes in bond vibration between the ground state and the TS of an enzyme catalysed reaction (reviewed by Schramm in 20186).
The process typically commences with the preparation of the substrates of the targeted enzyme incorporating stable isotopes (e.g. 18O, 15N, 14C, 13C, 3H and 2H) at chemically relevant positions (Fig. 1).5-6 Next, experimental conditions must be developed that are suitable to determine the intrinsic KIEs, that is, isotope effects relating only to the chemical step. Following the measurement of the KIEs, the values can be matched to theoretical values obtained using quantum mechanical calculations to obtain an image of the TS. Finally, chemically stable analogues mimicking the features of the TS (e.g. bond lengths, bond angles and charges) can be designed, synthesised and tested against the target enzyme(s).
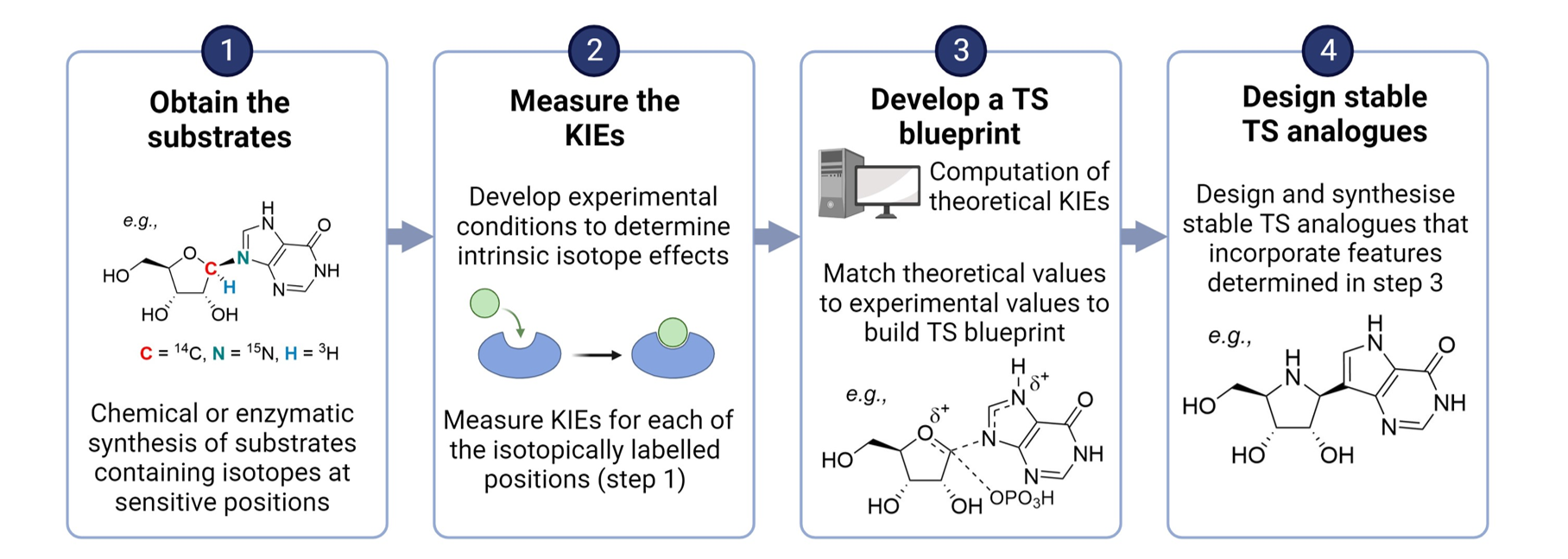
Immucillin H (Forodesine, BCX-1777, the active ingredient of Mundesine®)
The invention of immucillin H (Mundesine®, Forodesine, BCX-1777) is arguably the greatest success story coming out of the enzyme inhibitor programme at the Ferrier Research Institute, having gained approval in Japan for use against relapsed/refractory peripheral T-cell lymphoma.12 In fact, immucillin H was only the second drug born out of a New Zealand laboratory and translated to the international market.13-14 Immucillin H targets purine nucleoside phosphorylase (PNP), an important enzyme of the purine salvage pathway, catalysing the reversible phosphorolysis of ribose and deoxyribose nucleosides.15 PNP is required for purine degradation, and its absence leads to deoxy-guanosine triphosphate (dGTP) accumulation.16 High levels of dGTP are thought to inhibit ribonucleotide diphosphate reductase, restricting DNA replication and, therefore, the expansion of T-cell populations.5 As the rapid proliferation of T-cells is a hallmark of several diseases (e.g. T-cell lymphomas and autoimmune diseases), PNP is a highly attractive target for the design of enzyme inhibitors.
Early efforts to develop potent PNP inhibitors yielded several candidates, each with nanomolar inhibitory constants against human PNP.5 The most noteworthy example is a guanine analogue developed by BioCryst Pharmaceuticals, known as Peldesine (BCX-34).17-18 Peldesine demonstrated a nanomolar IC50 against human PNP and was subsequently progressed to human clinical trials, with the primary focus of studies being the assessment of Peldesine against psoriasis19 and cutaneous T-cell lymphoma.20 Peldesine, however, showed limited effectiveness in clinical trials, often attributed to its fast off-rate, which hinders adequate PNP inhibition.5, 21-22
To advance efforts towards a clinically efficacious PNP inhibitor, the Ferrier Research Institute collaborated with Prof. Schramm at the Albert Einstein College of Medicine and applied their transition state analysis drug design approach to PNP. Reasoning that the bovine enzyme bore significant similarity to the human enzyme (87% sequence identity), the TS of bovine PNP was first employed to inform TS analogue design.23-24 KIE measurements were used to analyse the bovine enzyme TS, which confirmed a relatively early TS with significant bond order to the outgoing nucleobase (e.g. bond length of 1.8 Å) and limited involvement of the incoming nucleophile (e.g. bond length of 3.0 Å, Fig. 2a).23 Additionally, these results indicated the presence of a ribooxocarbenium ion and protonation of the nucleobase (N-7) at the TS. From this blueprint, immucillin H (Fig. 2b) was designed and consisted of an iminoribitol group, which, when protonated, mimics the positive charge observed at the TS.25-26 Furthermore, incorporation of the 9-deazahypoxanthine group in place of the hypoxanthine moiety removed the possibility of phosphorolysis while simultaneously increasing the pKa at N-7 of the nucleobase. Biological analysis of immucillin H revealed potent inhibitory activity, with dissociation constants (Ki*) of 23 pM26 against bovine PNP and 56 pM27 against human PNP, respectively. Additional pre-clinical assessment of immucillin H showcased the inhibitor’s excellent bioavailability properties and ability to induce cell death in malignant T-cell leukaemia cell lines.8, 22, 28
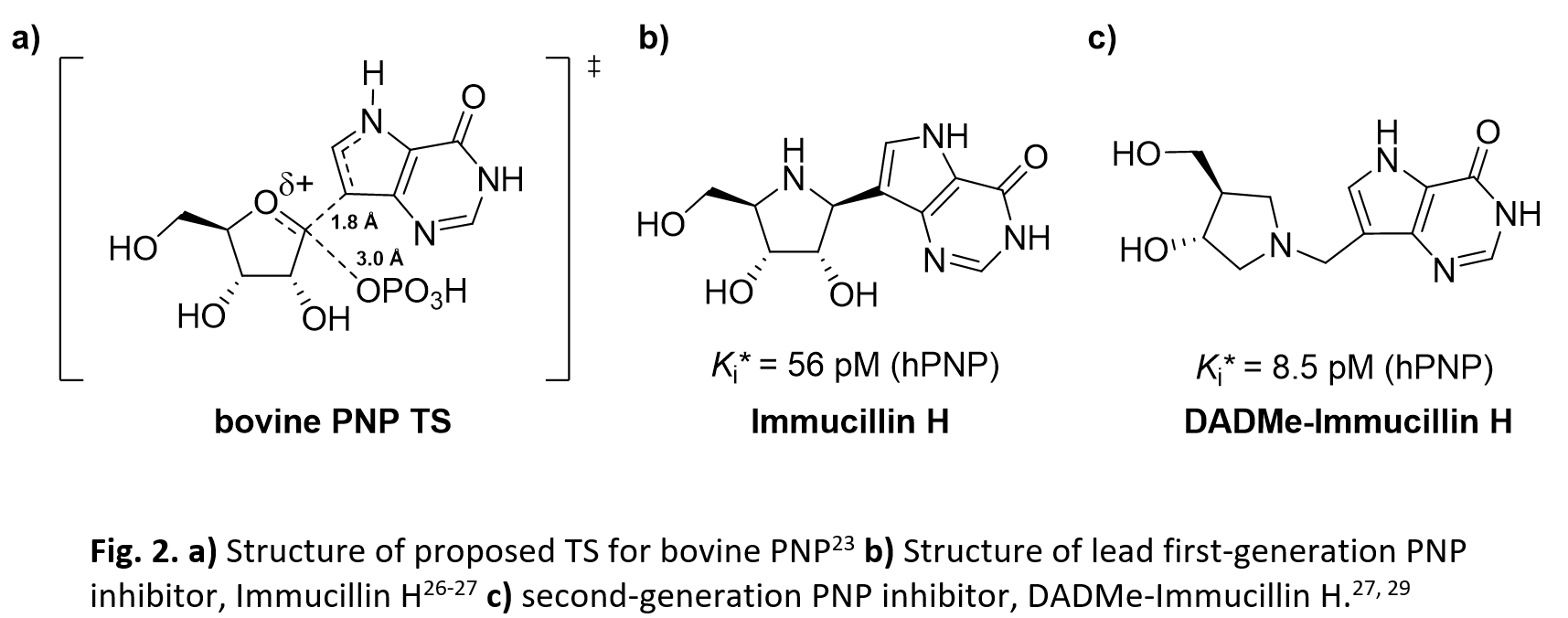
Despite the sequence similarity between human and bovine PNP, there are significant differences in the nature of the TS.24 While the bovine enzyme features an early TS, with significant bond order to the nucleobase, the TS of the human enzyme contains a significant positive charge at the anomeric carbon and a long bond length (3.0 Å) to the outgoing nucleobase.30 Accordingly, a second generation of PNP TS analogues was designed and synthesised, including DAD-Me-Immucillin H (Fig. 2c).27 To most accurately mimic the dissociated ribocation, DADMe-Immucillin H incorporates a tertiary amine at the pseudo-anomeric position, while the hydroxyl at the 2-position was removed to ensure compound stability. Together, these alterations better mimic the TS and resulted in a significant decrease in inhibition constant. Third- and fourth-generation TS analogues simplified the inhibitor structure, easing the synthetic burden while retaining affinity for the enzyme.5, 24, 31
Given the pre-clinical success of immucillin analogues against PNP, BioCryst Pharmaceuticals licensed a subset of the synthesised analogues and instigated human clinical trials.5 Here, immucillin H (under the tradename Forodesine) was assessed against various T-cell and B-cell malignancies, with additional trials carried out against cutaneous T-cell lymphoma.21-22, 32-33 The oral administration of immucillin H was found to be tolerated, with few drug-related serious adverse effects reported.22, 34 Furthermore, sufficient clinical efficacy was observed. Following the sublicensing of immucillin H to Mundipharma (under the tradename Mundesine®), immucillin H received approval in Japan for the treatment of relapsed/refractory peripheral T-cell lymphoma.5, 12 Surprisingly, the clinical efficacy of immucillin H was only observed in a subset of patients (approximately 25%). In 2020, Rehwinkel and co-workers provided a potential explanation by elegantly demonstrating that an additional enzyme, a phosphohydrolase known as SAMHD1, protects cells against immucillin H-induced cell death.35 Here, SAMHD1 was thought to protect against dGTP imbalances by converting dGTP into deoxyguanosine. As a proportion of chronic lymphocytic patients acquire loss-of-function mutations in SAMHD1, the efficacy of immucillin H may be limited to this group of patients.
Targeting malaria with TS analogues and acyclic derivatives
Over the last 25 years, the TS technology has been employed to design inhibitors targeting the protozoan parasite Plasmodium falciparum (P. falciparum). Infection with P. falciparum, the causative agent of malaria, represents an immense global health burden, and increasing levels of pharmaceutical resistance are causing mounting concern.36-37 P. falciparum is a purine auxotroph, lacking the machinery necessary for de novo synthesis of purines and instead relies on the purine salvage pathway to convert materials scavenged from erythrocytes into purine nucleotides and nucleic acids.37 Hypoxanthine-guanine-xanthine (HGX) phosphoribosyltransferase (PRT) is an essential enzyme of the purine salvage pathway, converting purine bases into the corresponding purine nucleotides (scheme 1). Given the reliance of P. falciparum on HGXPPRT, HGXPRT represents an attractive target for the selective elimination of P. falciparum. Accordingly, many reports have been published on the generation of inhibitors targeting this important enzyme (for a selection of examples, please see the following references38-53).
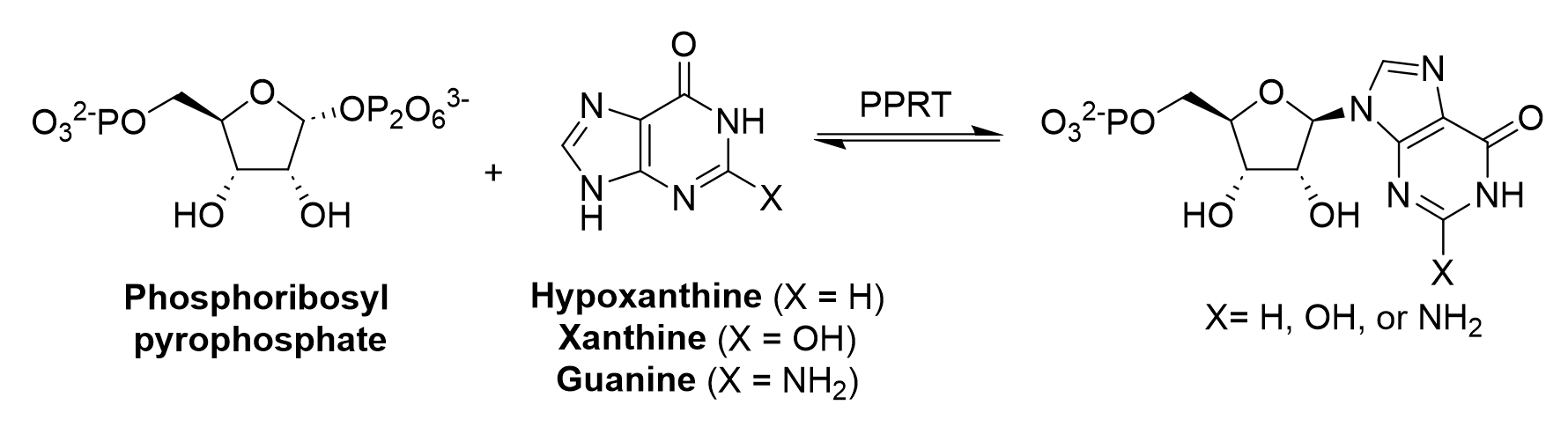
To create a blueprint of the TS of the HGXPRT catalysed reaction, Schramm’s team embarked on an arduous journey. In this case, no TS structure of a PRT-catalysed reaction using isotopically labelled phosphoribosyl pyrophosphate (PRPP) had been reported in the physiological direction.54 In lieu, several TSs of PRT-catalysed reactions had been reported in the reverse direction,55-56 that is, the PRT-catalysed pyrophosphorolysis of the reaction product. This lack of data was likely due to the hydrolytic instability of PRPP, the reaction substrate, of which labelled analogues are required for KIE measurements. Schramm’s team used an enzymatic route to prepare the necessary isotopically labelled analogues of PRPP, which required rapid purification under mild conditions to avoid substrate degradation.54 KIE measurements and subsequent computational efforts yielded a map of the TS for the HGXPRT-catalysed formation of inosine monophosphate. Here, the data suggested that the reaction proceeded with a SN1-like TS, with a significant positive charge at the anomeric centre (Fig. 3a). Bond contributions from the incoming hypoxanthine base and outgoing pyrophosphate group were asymmetric (bond orders of 0.205 and 0.142, respectively), suggesting that the TS was more product-like than substrate-like.
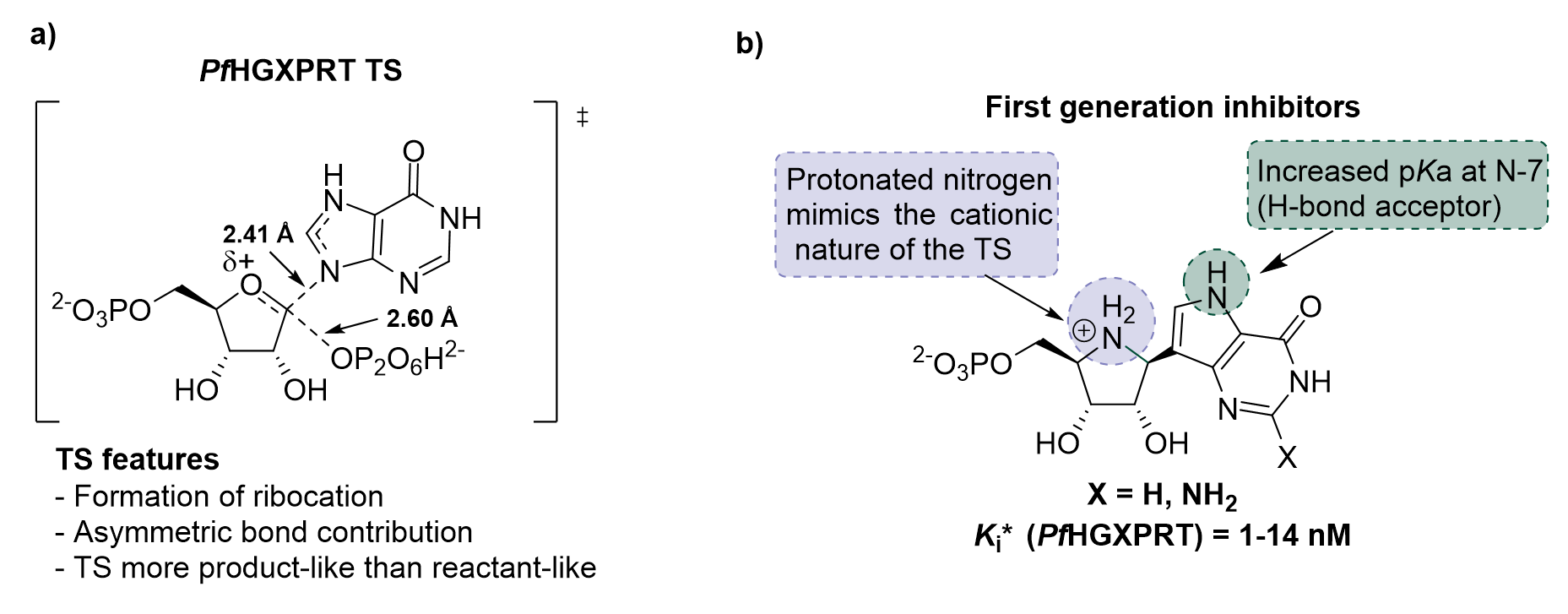
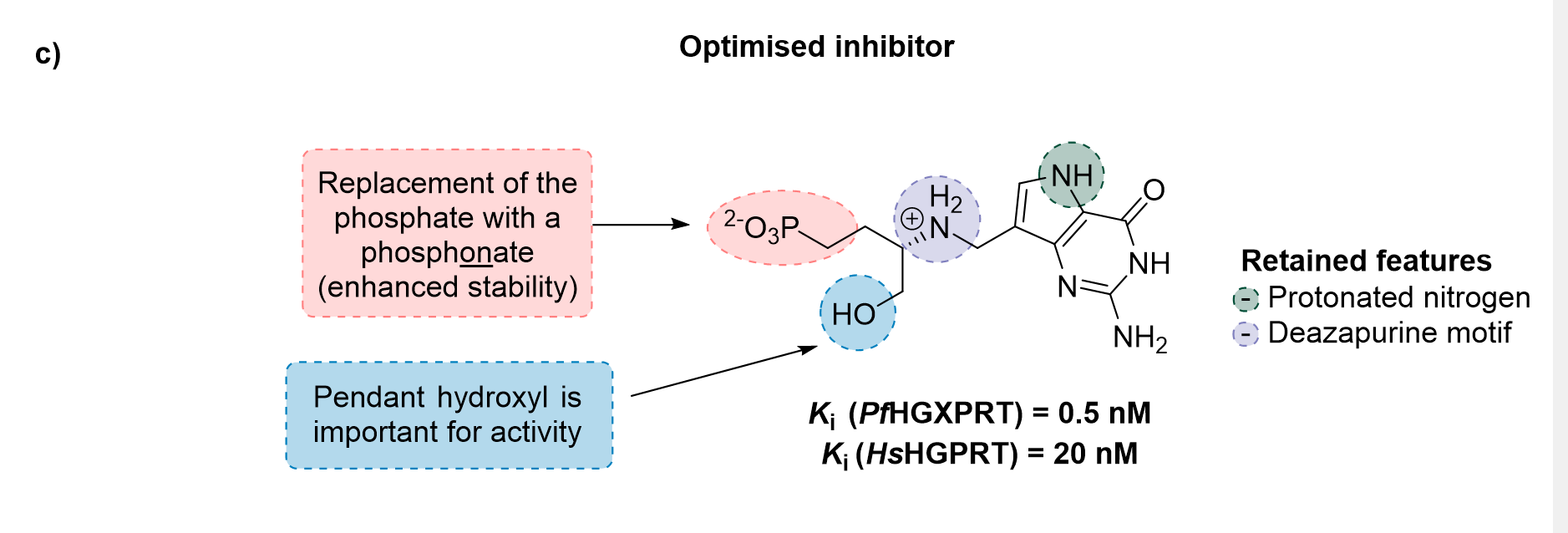
The first-generation inhibitors created by the Ferrier Research Institute were structurally related to the potent PNP inhibitor, immucillin H. In a similar manner to immucillin H, the protonated endocyclic nitrogen serves as a mimic of the positive charge formed at the TS. Furthermore, the incorporation of a 9-deazapurine group functioned to eliminate the possibility of pyrophosphorolysis while increasing basicity at N-7 of the purine ring (Fig. 3b).52 In contrast to PNP, however, a 5ʹ-phosphate was required for inhibitory activity against PfHGXPRT, with the lead candidates providing Ki values of 1 – 14 nM. Further research served to simplify the inhibitor scaffold, thereby providing valuable insight into the structure-activity-relationship and a reduction in the overall synthetic burden.40 Here, a selection of acyclic nucleoside phosphates and phosphonates were prepared and evaluated for their ability to inhibit PfHGXPRT. One promising inhibitor incorporated a secondary nitrogen bound through a 3-carbon linker to a terminal phosphonate (Fig. 3c) - inclusion of a terminal phosphonate in place of a phosphate offered enhanced chemical stability. Additionally, the incorporation of a pendent hydroxymethyl group was found to be important to overall inhibitory activity. The optimised structure inhibited PfHGXPRT with an inhibitory constant of 0.5 nM and showed selectivity over human HGPRT (selectivity index of 40).39
While the lead structure showed potent inhibitory activity against the purified enzyme, the free phosphonate exhibited poor activity in cellular models (e.g., cultured parasites), likely due to a lack of cell membrane permeability.42 Accordingly, several prodrugs of the lead compound have been prepared that show enhanced activity in in vitro and in vivo models of malaria. Efforts to produce more efficacious prodrugs of the lead compound are currently underway.
Targeting Clostridium difficile TcdA and TcdB toxins with TS analogues
Given the mounting concerns surrounding global antibiotic resistance,57 the teams at the Ferrier Research Institute and Albert Einstein College of Medicine have commenced work on adapting the TS analogue platform for the generation of anti-toxins agents. These anti-toxins are intended to replace antibiotics without the harmful effects of gut disruption caused by repeated antibiotic therapies.
In recent years, the teams have targeted infection by Clostridioides difficile (C. difficile), a spore-forming, gram-positive bacteria that can induce symptoms varying from mild diarrhoea to severe inflammatory disease of the colon.58C. difficile infections typically arise in patients who have received treatment with broad-spectrum antibiotics, leading to an imbalance of the gastrointestinal microbiota that permits C. difficile spores to proliferate and colonise the GI tract. C. difficile produces a set of toxins, toxins A (TcdA), B (TcdB), and transferase toxin (CDT or binary toxin), which are the major determinants of C. difficile-related pathology59 and have been thoroughly reviewed elsewhere.58, 60 Briefly, TcdA and TcdB are glucosylating toxins comprised of at least four different functional domains, including a glucosyltransferase domain (GTD). These toxins can catalyse the transfer of a glucose unit from uridine diphosphate glucose (UDP-glucose) onto host Rho family guanosine triphosphatases (GTPases, Scheme 2). Glucosylation of Rho family GTPases disrupts the organisation of the cytoskeleton and induces inflammation and apoptosis. In the absence of a suitable acceptor, TcdA and TcdB can catalyse the hydrolysis of UDP-glucose into UDP and free glucose.61 While TcdA and TcdB are the major toxins responsible for C. difficile-related pathology, CDT is produced by some clinical isolates of C. difficile (5-30% of isolates) and can contribute to disease.
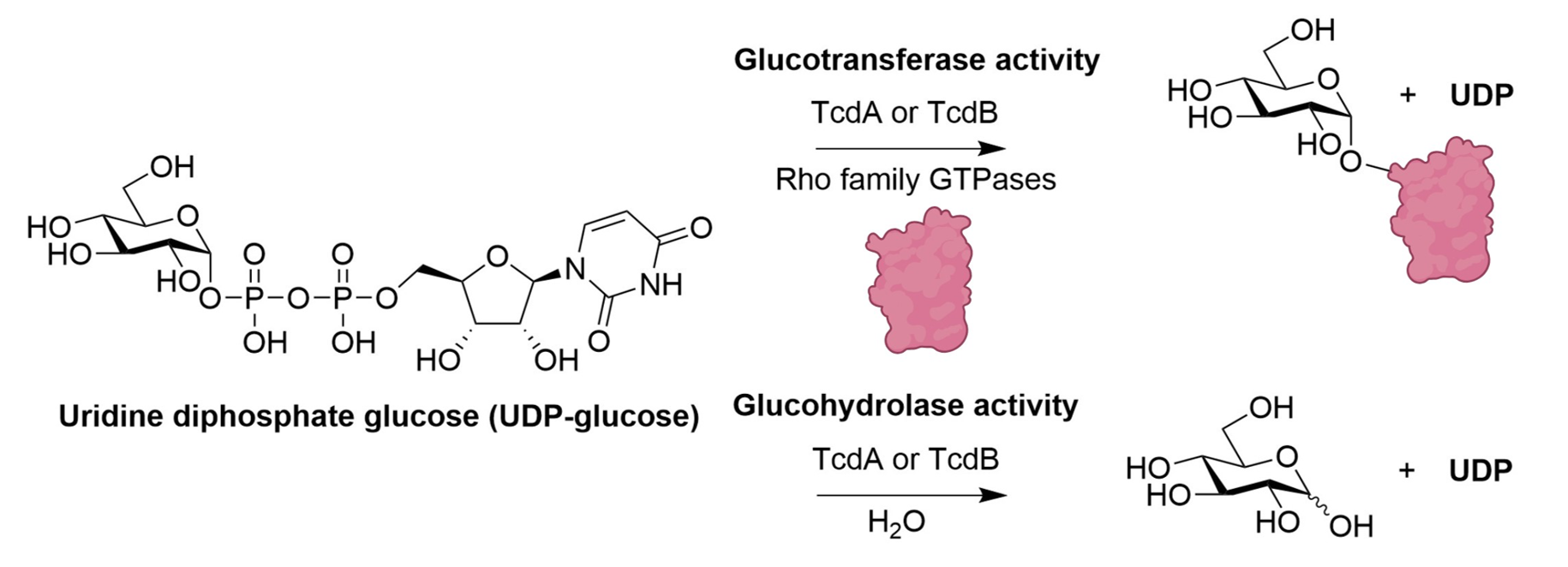
At present, there are several options used to treat C. difficile infections, with the administration of antibiotics that are effective against C. difficile infections (e.g., vancomycin or fidaxomicin) preferred in the majority of cases.63 However, the use of antibiotics can arrest restoration of the normal gut microbiota, and resultingly, recurrence of C. difficile infection occurs in approximately one-fifth of patients. Given the importance of the C. difficile toxins TcdA and TcdB to pathology, there has been significant interest in targeting these toxins. Indeed, a range of small molecules and biologics targeting these toxins have been reported in recent years.64-72 Of particular note is Bezlotoxumab, a human monoclonal antibody that targets TcdB.72 Bezlotoxumab was approved for use in the United States in patients receiving antibiotic treatment for CDI and who are considered at high risk of recurrent infections. The use of antibodies, however, may be highly specific to a single toxin and variant of that toxin, and it is therefore of interest to develop small molecule anti-toxins that broadly target TcdA and TcdB.
To develop potent, small molecule inhibitors of the TcdA and TcdB toxins, Schramm’s team employed a range of techniques to probe the mechanism and TS of the glucosyltransferase activity of TcdB73 and the glucohydrolase activity of TcdA and TcdB.62 On the basis of KIE experiments, it was inferred that TcdA and TcdB catalysed the hydrolysis of UDP-glucose, forming a TS with significant positive charge and sp2 character at the anomeric centre.62 The glucose moiety, which would typically sit in a chair conformation, was thought to be flattened, with C-5, the endocyclic oxygen, C-1, and C-2 residing in a near co-planar arrangement. In a second publication, Schramm and co-workers studied the glucosyltransferase activity of TcdB.73 Through a combination of kinetic, KIE, and positional isotope exchange (PIX) experiments, it was found that TcdB glucosylated the Rho GTPase, Rac1, with retention of stereochemistry via an ‘internal return’ SNi-like mechanism. Furthermore, KIE measurements supported the presence of an oxocarbenium ion pair at the TS, with the sugar occupying a boat conformation (B2,5, Fig. 3a).

Iminosugars are sugar analogues that incorporate a nitrogen atom in place of the endocyclic oxygen.74 Iminosugars have been extensively investigated as inhibitors of glycosylhydrolases and are thought to operate by mimicking the glycocationic TS.75 Here, the endocyclic nitrogen is often protonated at physiological pH, which is a suitable mimic for TSs that contain positive charges at the endocyclic oxygen or anomeric centre. The iminosugars isofagomine and noeuromycin are known glucosidase inhibitors that retain the gluco-configuration but incorporate a nitrogen atom in place of the anomeric carbon.
Given the structural similarities between these iminosugars and the proposed TS of TcdA and TcdB glucosyltransferases, isofagomine and noeuromycin were investigated for their ability to inhibit these glucosyltransferases.62 Isofagomine and noeuromycin inhibited TcdA GTD with inhibition constants of 0.24 ± 0.01 μM and 4.7 ± 0.2 μM (respectively) and TcdB GTD with inhibition constants of 1.4 ± 0.1 μM and 10.6 ± 1.0 μM (respectively). Isothermal titration calorimetry experiments and crystal structures of inhibitor complexes indicated that the iminosugars form a ternary complex with the enzyme and UDP. Accordingly, kinetic assays were carried out to inhibit TcdA-GTD and TcdB-GTD glucosyltransferase activity in the presence of a fixed concentration of UDP. Here, inhibitory affinity was significantly improved, with isofagomine and noeuromycin providing inhibitory constants of 16 ± 2 nM and 210 ± 50 nM (respectively) against TcdA-GTD. Finally, in vitro testing of isofagomine and noeuromycin using cell rounding assays and western blot analysis of Rac1 provided evidence that these iminosugars could prevent toxin-induced cytotoxicity in Vero cells. While work towards TcdA-GTD and TcdB-GTD inhibitors is ongoing, the work published to date has demonstrated the potential of iminosugars, such as isofagomine and noeuromycin, as small molecule inhibitors of C. difficile toxins.
Concluding remarks
Compounds incorporating the structural and electronic properties of the TS of an enzyme-catalysed reaction often deliver highly potent and specific enzyme inhibitors by forming a tightly bound and thermodynamically stable complex with the enzyme.6 Accordingly, it is of particular interest to understand the nature of the TS of pharmaceutically relevant enzymes. Over the course of the last three decades, the Ferrier Research Institute has worked with Professor Vern Schramm to refine the process of mapping the TS of enzyme-catalysed reactions and converting that information into chemically stable mimics.
The examples described in this article represent a brief yet crucial glimpse into the remarkable collaborative efforts between the chemists at the Ferrier Research Institute and their long-standing collaborator at the Albert Einstein College of Medicine in New York, USA. For additional examples, interested readers are encouraged to explore Schramm’s comprehensive review on ‘enzymatic transition states and drug design’.6
While the research presented here is primarily focused on enzymes with ribocationic transition states, the TS technology is applicable to a variety of targets. It is, however, no coincidence that ribocationic transition states have featured so heavily in this article. Ribotransferases embody many qualities needed for successful TS analogue design, including altered geometry, hybridization, and charge distribution at the TS.11 Undoubtedly, this technology will continue to be applied across a wide range of enzymes for drug discovery .
References
- de Azevedo Jr, W.F.; Canduri, F.; dos Santos, D.M.; Silva, R.G.; de Oliveira, J.S.; de Carvalho, L.P.S.; Basso, L.A.; Mendes, M.A.; Palma, M.S.; Santos, D.S. Biochemical and Biophysical Research Communications 2003, 308, 545-552.
- Copeland, R.A. Evaluation of enzyme inhibitors in drug discovery: a guide for medicinal chemists and pharmacologists, John Wiley & Sons, 2nd ed. 2013.
- Radzicka, A.; Wolfenden, R. Science 1995, 267, 90-93.
- Evans, G.B.; Tyler, P.C.; Schramm, V.L. ACS infectious diseases 2018, 4, 107-117.
- Evans, G.B.; Schramm, V.L.; Tyler, P.C. Medchemcomm 2018, 9, 1983-1993.
- Schramm, V.L. Chemical Reviews 2018, 118, 11194-11258.
- Schramm, V.L. ACS chemical biology 2013, 8, 71-81.
- Kicska, G.A.; Long, L.; Hörig, H.; Fairchild, C.; Tyler, P.C.; Furneaux, R.H.; Schramm, V.L.; Kaufman, H.L. Proceedings of the National Academy of Sciences 2001, 98, 4593-4598.
- Pauling, L. American Scientist 1948, 36, 51-58.
- Murkin, A.S.; Schramm, V.L. Drug Design: Structure-and Ligand-Based Approaches 2010, 215-247.
- Schramm, V.L. Annual review of biochemistry 2011, 80, 703-732.
- BioCryst Pharmaceuticals BioCryst Announces Mundipharma Receives Approval for Mundesine® in Japan 2017, https://biocryst.gcs-web.com/news-releases/news-release-details/biocryst-announces-mundipharma-receives-approval-mundesiner (accessed 16/10/2023).
- Reidy, M. Cancer cure 20 years in the making hits international shelves, Stuff, July 23, 2017, https://www.stuff.co.nz/business/innovation/94806030/cancer-cure-20-years-in-the-making-hits-international-shelves (accessed 25/10/2023).
- Royal Society Te Apārangi 2017 MacDiarmid Medal: Novel drug design technology leads to new lymphoma drug 10 October, 2017, https://www.royalsociety.org.nz/what-we-do/medals-and-awards/medals-and-awards-news/macdiarmid-medal-novel-drug-design-technology-leads-to-new-lymphoma-drug/ (accessed 25/10/2023).
- Bzowska, A.; Kulikowska, E.; Shugar, D. Pharmacology & Therapeutics 2000, 88, 349-425.
- Fairbanks, L.D.; Taddeo, A.; Duley, J.A.; Simmonds, H.A. Journal of Immunology 1990, 144, 485-491.
- Ealick, S.E.; Babu, Y.S.; Bugg, C.E.; Erion, M.D.; Guida, W.C.; Montgomery, J.A.; Secrist 3rd, J. Proceedings of the National Academy of Sciences 1991, 88, 11540-11544.
- Bantia, S.; Montgomery, J.A.; Johnson, H.G.; Walsh, G.M. Immunopharmacology 1996, 35, 53-63.
- Morris Jr, P.E.; Omura, G.A. Current Pharmaceutical Design 2000, 6, 943-959.
- Duvic, M.; Olsen, E.A.; Omura, G.A.; Maize, J.C.; Vonderheid, E.C.; Elmets, C.A.; Shupack, J.L.; Demierre, M.F.; Kuzel, T.M.; Sanders, D.Y. Journal of the American Academy of Dermatology 2001, 44, 940-947.
- Gandhi, V.; Kilpatrick, J.M.; Plunkett, W.; Ayres, M.; Harman, L.; Du, M.; Bantia, S.; Davisson, J.; Wierda, W.G.; Faderl, S. Blood 2005, 106, 4253-4260.
- Al-Kali, A.; Gandhi, V.; Ayoubi, M.; Keating, M.; Ravandi, F. Future oncology 2010, 6, 1211-1217.
- Kline, P.C.; Schramm, V.L. Biochemistry 1993, 32, 13212-13219.
- Ho, M.-C.; Shi, W.; Rinaldo-Matthis, A.; Tyler, P.C.; Evans, G.B.; Clinch, K.; Almo, S.C.; Schramm, V.L. Proceedings of the National Academy of Sciences 2010, 107, 4805-4812.
- Evans, G.B.; Furneaux, R.H.; Gainsford, G.J.; Schramm, V.L.; Tyler, P.C. Tetrahedron 2000, 56, 3053-3062.
- Miles, R.W.; Tyler, P.C.; Furneaux, R.H.; Bagdassarian, C.K.; Schramm, V.L. Biochemistry 1998, 37, 8615-8621.
- Evans, G.B.; Furneaux, R.H.; Lewandowicz, A.; Schramm, V.L.; Tyler, P.C. Journal of Medicinal Chemistry 2003, 46, 5271-5276.
- Bantia, S.; Ananth, S.L.; Parker, C.D.; Horn, L.L.; Upshaw, R. International Immunopharmacology 2003, 3, 879-887.
- Taylor, E.A.; Clinch, K.; Kelly, P.M.; Li, L.; Evans, G.B.; Tyler, P.C.; Schramm, V.L. Journal of the American Chemical Society 2007, 129, 6984-6985.
- Lewandowicz, A.; Schramm, V.L. Biochemistry 2004, 43, 1458-1468.
- Clinch, K.; Evans, G.B.; Fröhlich, R.F.; Furneaux, R.H.; Kelly, P.M.; Legentil, L.; Murkin, A.S.; Li, L.; Schramm, V.L.; Tyler, P.C. Journal of Medicinal Chemistry 2009, 52, 1126-1143.
- Dummer, R.; Duvic, M.; Scarisbrick, J.; Olsen, E.A.; Rozati, S.; Eggmann, N.; Goldinger, S.M.; Hutchinson, K.; Geskin, L.; Illidge, T.M.; Giuliano, E.; Elder, J.; Kim, Y.H. Annals of Oncology 2014, 25, 1807-1812.
- Duvic, M.; Forero-Torres, A.; Foss, F.; Olsen, E.A.; Kim, Y. Blood 2006, 108, 2467.
- Dummer, R.; Duvic, M.; Scarisbrick, J.; Olsen, E.; Rozati, S.; Eggmann, N.; Goldinger, S.; Hutchinson, K.; Geskin, L.; Illidge, T. Annals of Oncology 2014, 25, 1807-1812.
- Davenne, T.; Klintman, J.; Sharma, S.; Rigby, R.E.; Blest, H.T.; Cursi, C.; Bridgeman, A.; Dadonaite, B.; De Keersmaecker, K.; Hillmen, P. Cell reports 2020, 31.
- World Health Organization, Malaria 2023, https://www.who.int/news-room/fact-sheets/detail/malaria (accessed 01/11/2023).
- Cheviet, T.; Lefebvre-Tournier, I.; Wein, S.; Peyrottes, S. Journal of Medicinal Chemistry 2019, 62, 8365-8391.
- Kaiser, M.M.; Hocková, D.; Wang, T.H.; Dračínský, M.; Poštová‐Slavětínská, L.; Procházková, E.; Edstein, M.D.; Chavchich, M.; Keough, D.T.; Guddat, L.W. ChemMedChem 2015, 10, 1707-1723.
- VT Minnow, Y.; Suthagar, K.; Clinch, K.; Ducati, R.G.; Ghosh, A.; Buckler, J.N.; Harijan, R.K.; Cahill, S.M.; Tyler, P.C.; Schramm, V.L. ACS chemical biology 2022, 17, 3407-3419.
- Clinch, K.; Crump, D.R.; Evans, G.B.; Hazleton, K.Z.; Mason, J.M.; Schramm, V.L.; Tyler, P.C. Bioorganic & Medicinal Chemistry 2013, 21, 5629-5646.
- Hockova, D.; Keough, D.T.; Janeba, Z.; Wang, T.-H.; de Jersey, J.; Guddat, L.W. Journal of Medicinal Chemistry 2012, 55, 6209-6223.
- Hazleton, Keith Z.; Ho, M.-C.; Cassera, Maria B.; Clinch, K.; Crump, Douglas R.; Rosario, I.; Merino, Emilio F.; Almo, Steve C.; Tyler, Peter C.; Schramm, Vern L. Chemistry & Biology 2012, 19, 721-730.
- Hocková, D.; Holý, A.; Masojídková, M.; Keough, D.T.; de Jersey, J.; Guddat, L.W. Bioorganic & Medicinal Chemistry 2009, 17, 6218-6232.
- Keough, D.T.; Hockova, D.; Holy, A.; Naesens, L.M.; Skinner-Adams, T.S.; Jersey, J.d.; Guddat, L.W. Journal of Medicinal Chemistry 2009, 52, 4391-4399.
- Špaček, P.; Keough, D.T.; Chavchich, M.; Dračínský, M.; Janeba, Z.; Naesens, L.; Edstein, M.D.; Guddat, L.W.; Hocková, D. Bioorganic & Medicinal Chemistry 2017, 25, 4008-4030.
- Spacek, P.; Keough, D.T.; Chavchich, M.; Dracinsky, M.; Janeba, Z.; Naesens, L.; Edstein, M.D.; Guddat, L.W.; Hockova, D. Journal of Medicinal Chemistry 2017, 60, 7539-7554.
- Keough, D.T.; Hockova, D.; Janeba, Z.; Wang, T.-H.; Naesens, L.; Edstein, M.D.; Chavchich, M.; Guddat, L.W. Journal of Medicinal Chemistry 2015, 58, 827-846.
- Česnek, M.; Hocková, D.; Holý, A.; Dračínský, M.; Baszczyňski, O.; de Jersey, J.; Keough, D.T.; Guddat, L.W. Bioorganic & Medicinal Chemistry 2012, 20, 1076-1089.
- Klejch, T.; Pohl, R.; Janeba, Z.; Sun, M.; Keough, D.T.; Guddat, L.W.; Hocková, D. Tetrahedron 2018, 74, 5886-5897.
- Opoku, F.; Govender, P.P.; Pooe, O.J.; Simelane, M.B. Biomolecules 2019, 9, 861.
- Vaňková, K.; Keough, D.T.; Hocková, D.; Guddat, L.W.; Janeba, Z. ChemMedChem 2023, 18, e202300211.
- Li, C.M.; Tyler, P.C.; Furneaux, R.H.; Kicska, G.; Xu, Y.; Grubmeyer, C.; Girvin, M.E.; Schramm, V.L. Nature Structural Biology 1999, 6, 582-587.
- Shi, W.; Li, C.M.; Tyler, P.C.; Furneaux, R.H.; Grubmeyer, C.; Schramm, V.L.; Almo, S.C. Nature Structural Biology 1999, 6, 588-593.
- Ducati, R.G.; Firestone, R.S.; Schramm, V.L. Biochemistry 2017, 56, 6368-6376.
- Tao, W.; Grubmeyer, C.; Blanchard, J.S. Biochemistry 1996, 35, 14-21.
- Burgos, E.S.; Vetticatt, M.J.; Schramm, V.L. Journal of the American Chemical Society 2013, 135, 3485-3493.
- World Health Organization, Antibiotic resistance 2020, https://www.who.int/news-room/fact-sheets/detail/antibiotic-resistance (accessed 31/10/2023).
- Aktories, K.; Schwan, C.; Jank, T. Annual Review of Microbiology 2017, 71, 281-307.
- Kelly, C.P.; LaMont, J.T. New England Journal of Medicine 2008, 359, 1932-1940.
- Gerding, D.N.; Johnson, S.; Rupnik, M.; Aktories, K. Gut microbes 2014, 5, 15-27.
- Ciesla, W.P.; Bobak, D.A. Journal of Biological Chemistry 1998, 273, 16021-16026.
- Paparella, A.S.; Aboulache, B.L.; Harijan, R.K.; Potts, K.S.; Tyler, P.C.; Schramm, V.L. Nature Communications 2021, 12, 6285.
- Leffler, D.A.; Lamont, J.T. New England Journal of Medicine 2015, 372, 1539-1548.
- Bender, K.O.; Garland, M.; Ferreyra, J.A.; Hryckowian, A.J.; Child, M.A.; Puri, A.W.; Solow-Cordero, D.E.; Higginbottom, S.K.; Segal, E.; Banaei, N. Science Translational Medicine 2015, 7, 306ra148-306ra148.
- Letourneau, J.J.; Stroke, I.L.; Hilbert, D.W.; Cole, A.G.; Sturzenbecker, L.J.; Marinelli, B.A.; Quintero, J.G.; Sabalski, J.; Li, Y.; Ma, L. Bioorganic & medicinal chemistry letters 2018, 28, 3601-3605.
- Tam, J.; Beilhartz, G.L.; Auger, A.; Gupta, P.; Therien, A.G.; Melnyk, R.A. Chemistry & Biology 2015, 22, 175-185.
- Letourneau, J.J.; Stroke, I.L.; Hilbert, D.W.; Sturzenbecker, L.J.; Marinelli, B.A.; Quintero, J.G.; Sabalski, J.; Ma, L.; Diller, D.J.; Stein, P.D. Bioorganic & Medicinal Chemistry Letters 2018, 28, 756-761.
- Tam, J.; Hamza, T.; Ma, B.; Chen, K.; Beilhartz, G.L.; Ravel, J.; Feng, H.; Melnyk, R.A. Nature Communications 2018, 9, 5233.
- Korbmacher, M.; Fischer, S.; Landenberger, M.; Papatheodorou, P.; Aktories, K.; Barth, H. Frontiers in Pharmacology 2020, 11, 1204.
- Papatheodorou, P.; Kindig, S.; Badilla-Lobo, A.; Fischer, S.; Durgun, E.; Thuraisingam, T.; Witte, A.; Song, S.; Aktories, K.; Chaves-Olarte, E. Frontiers in Microbiology 2021, 12, 784856.
- Barthold, L.; Heber, S.; Schmidt, C.Q.; Gradl, M.; Weidinger, G.; Barth, H.; Fischer, S. International Journal of Molecular Sciences 2022, 23, 4509.
- Markham, A. Drugs 2016, 76, 1793-1798.
- Paparella, A.S.; Cahill, S.M.; Aboulache, B.L.; Schramm, V.L. ACS chemical biology 2022, 17, 2507-2518.
- Conforti, I.; Marra, A. Organic & Biomolecular Chemistry 2021, 19, 5439-5475.
- Gloster, T.M.; Davies, G.J. Organic & Biomolecular Chemistry 2010, 8, 305-320.